Wnt Signaling in Lymphopoiesis and Hematopoiesis
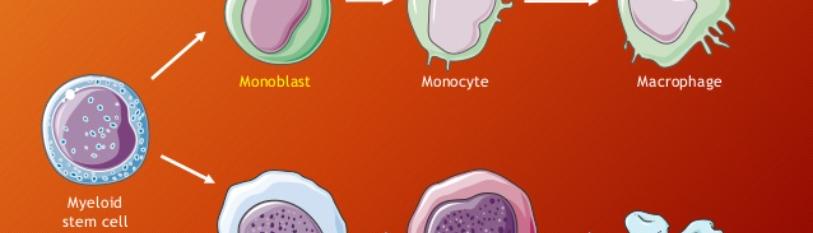
Imen Jridi, Karin Pike-Overzet, Kirsten Cante-Barrett and Frank JT Staal*
Department of Immunology, Leiden University Medical Center, Leiden, The Netherlands
*Corresponding author: Frank JT Staal, Department of Immunology, Leiden University Medical Center, Leiden, The Netherlands
Citation: Jridi I, Pike-Overzet K, Cante K, Staal FJT (2020) Wnt Signaling in Lymphopoiesis and Hematopoiesis. J Stem Cell Res. 1(2):1-27.
Received: July 20, 2020, | Published: August 7, 2020
Copyright© 2020 genesis pub by Jridi I, et al. CC BY-NC-ND 4.0 DEED. This is an open-access article distributed under the terms of the Creative Commons Attribution-Non Commercial-No Derivatives 4.0 International License., This allows others distribute, remix, tweak, and build upon the work, even commercially, as long as they credit the authors for the original creation.
DOI: https://doi.org/10.52793/JSCR.2020.1(2)-07
Abstract
Wnt proteins are secreted glycoproteins important for embryonic development, stem cell-mediated homeostasis and blood cell formation. Even though the precise roles of both canonical and non-canonical Wnt pathways in the bone marrow and thymus are controversial, numerous studies highlight the critical role of Wnt family in proliferation, fate decision and differentiation of hematopoietic stem cells and thymic progenitors. Their dysregulation during one of those processes is responsible for certain developmental defects and could promote blood cancer and auto-immune diseases. Wnt signaling has been explored in a large number of primary research papers; however, there are a lot of questions to be addressed about the molecular mechanism and the down-stream genes that are involved in cell fate and the decision to differentiate in one specific lineage and not the other or to maintain naïve phenotypes. In this review, we focus on the relatively small number of studies that report on Wnt signaling in human hematopoiesis and lymphopoiesis. In order to discuss these studies, we first extensively review the different types of Wnt signaling pathways, their complexity and their cross-talk with β-catenin-mediated transcription. We discuss the most important Wnt signaling components and intracellular, cytoplasmic and nuclear signaling and feedback regulation. Finally, Wnt proteins in hematopoiesis are discussed with a particular attention to a comparison between mice and human.
Keywords
Wnt proteins; Lymphopoiesis; β-catenin; Wnt/β-catenin pathway; Threonine kinase
Introduction
Wnt proteins comprise a large family of highly conserved growth factors that tightly interact with extracellular matrix and bind to Frizzled on the cell surface [1]. This binding is promiscuous and the ligand/receptor specificities are not yet properly determined although some selectivity has become apparent. Depending on the type of the ligand-receptor interaction, the presence of intracellular signaling components and the target cell, two signaling pathways downstream of Wnt ligand receptor have been identified: the canonical and non-canonical pathways. The difference between these two pathways is that a canonical pathway involves β-catenin protein while a non-canonical pathway operates independently from it. The canonical Wnt pathway leads to the activation of Disheveled- proteins, which indirectly help to withhold the GSK-3β kinase activity, leading to a greater stability of β-catenin and activation of transcription via a complex formed with TCF/LEF transcription factors. However, the non-canonical pathway is initiated by Wnt binding to FZD receptor without stabilizing β-catenin. Instead, the down-stream signaling is mediated by other proteins. In the Ca2+-dependent Wnt pathway, it induces the increase of intracellular Ca2+ level, but, it could activate JNK pathway through Rho/Rac GTPases (Wnt/JNK pathway), and in the same manner, the increase of intracellular Ca2+ as a result of non-canonical Wnt signaling can lead to subsequent activation of several pathways including JNK, ROR, Yap/TAZ, FYN-STAT3 [2-3].
Wnt proteins are involved in different physiological processes such as embryonic development, apoptosis, cell growth regulation of adult tissues and hematopoiesis. In hematopoietic tissues, Wnt proteins are expressed in thymus epithelial cells, bone marrow osteoblasts, and hematopoietic stem cells (HSC). They ensure the communication between HSCs and stromal cells as well as with several other stem cell niches and play a key role in HSCs maturation into a specific lineage. It has been demonstrated that Wnt regulates the differentiation of HSC, myeloid precursors, and T lymphoid precursors during hematopoiesis [3-5]. However, different studies found controversial results about the role of Wnt in hematopoiesis. Some studies demonstrated that Wnt proteins could promote immune cells differentiation into more committed cells, while, other studies showed that Wnt could abrogate this differentiation and maintain their multipotency capacity [6-9]. The existence of many different Wnt proteins and their—sometimes opposite—functions hampers the study of Wnt signaling during hematopoiesis. Additionally, considerable species variation exists at very early stages of embryonic development, especially during gastrulation and morphogenesis; this precludes precise extrapolations from mouse to human in assigning specific roles for Wnt during early development. Thus, Wnt factors can be cell- and species dependent [9-10]. In this review, we summarize the members of the Wnt protein family, we explain in detail the different pathways, the molecular mechanism underlying each one of these, and how Wnt signaling is up- ordown regulated. We illustrate the different roles of Wnt proteins in blood cells with a particular focus on hematopoiesis, including B and T lymphopoiesis. Because of the rapid progress in this field, Wnt function in hematopoiesis is reviewed often, but most of what is known is from murine studies.In this review we focus on Wnt function in human hematopoiesis, pluripotency and cell fate and the comparison with these processes in mice. Despite the fact that Wnt signaling is very well conserved, certain aspects are unique in humans.
Wnt Family Key Players
-
Wnt
Wnt proteins belong to a large family of secreted glycosylated lipid-modified cysteine-rich proteins. So far, based on their amino-acid sequence rather than their functional properties, at least 19 members of Wnt (Table 1) and 10 different seven-pass transmembrane Frizzled receptors and co-receptors have been identified in mammals, including mice and humans [1-2]. Following synthesis and translocation to the endoplasmic reticulum (ER), Wnt proteins go through multiple post-translational modifications, the most important is palmitoylation on a conserved cysteine, shared between all Wnts. As this makes Wnts more hydrophobic and insoluble than initially predicted from the primary amino acid sequence, it makes very hard to purify them [11]. The enzyme responsible for this palmitoylation is a special palmitoyl transferase known as Porcupine [11-12].
Prot |
Role |
Structure |
Tissue |
Blood specificity |
Canonical/β-catenin pathway |
||||
Wnt1 |
- Growth factor for Double Negative Thymocytes in mice - Has a role in osteoblast function, bone development and bone homeostasis in human (71-79- 80) |
- Encoded by 4 exons and is clustered with WNT10B in a head-to-head manner within an interval of less than 7 kb and localized in chr12q13 in human and in chr15 56.8 in mice. |
Blood, brain, placenta |
MAIT T-cell, gdT-cell, memory CD8 T-cell, naive CD8 T-cell, memory CD4 T-cell |
Wnt2 |
- Produced by mouse macrophages (81) |
- Localized in chr 7q31 in human and in chr6 in mice. |
endometrium, lung, placenta |
Not detected in immune cells |
Wnt3 |
- Required for normal gastrulation and the formation of the primitive streak, the mesoderm during early embryogenesis, the apical ectodermal ridge, normal embryonic and limb development (82). |
- Comparison of the deduced mouse and human WNT3 protein sequences showed 4 changes in 333 amino acids and localized in chr 17q21 in human and in chr11 63 in mice |
liver, skin |
plasmacytoid DC |
Wnt3a |
- HSCs self-renew and differentiation to all lineages of the blood in mice. - Maintaining the self-renewing stem cell-like properties of mature memory CD8-positive T cells in mice and growth factor for Pro-B cells, Human CD34+ prothymocytes - Memory CD8 T-cell formation during immunization, resulting in enhanced immunity upon a second encounter with the same pathogen in mice (25-63-83) |
- Localized in chr1q42 in human and in chr11 32 in mice and encodes a deduced 352-amino acid protein containing an N-linked glycosylation site and residues conserved among members of the WNT family. - Shares 84.9% and 96% sequence identity with human and mice Wnt3, respectively. Wnt3a gene contains 4 exons and spans about 53 kb of genomic DNA. |
lung, placenta, spleen, and prostate |
Not detected in immune cells |
Wnt8a |
- Expressed by human bone marrow B-cell progenitor (83) |
- 6 exons of the Wnt8a gene encode a 351-amino acid protein with an N-terminal signal peptide, 3 N-linked glycosylation sites, and residues conserved among members of the WNT family. It shares 63.2% sequence identity with human Wnt8b and homology with the mouse Wnt8d protein. It is localized in chr5 in human and in chr18 in mice. |
brain, testis |
plasmacytoid DC |
Wnt8b |
- Not identified (84) |
- Wnt8b gene contains 6 exons separated by small introns, with the exception of intron 1. The predicted protein has 351 amino acids and localized in chr10q24 in human and in chr19 in mice. |
adrenal gland, parathyroid gland |
naive CD8 T-cell |
Wnt10a |
- Expressed by human bone marrow B-cell progenitors (3- 79) |
- Wnt10a gene contains 4 exons and is clustered with WNT6 in a head-to-tail manner with an interval of less than 7 kb and localized in chr2q35 in human and in chr1 in mice. |
Tongue, fetal kidney and lung, placenta, spleen, kidney, prostate, and ovary |
plasmacytoid DC |
Wnt10b |
- Growth factor for mouse fetal liver HSC and human CD34+ bone marrow cells - Produced by mouse macrophage (85) |
- The human Wnt10b sequence is 88% and 95% identical to the mouse gene at nucleotide and amino acid levels, respectively. It is encoded by 5 exons and is clustered with WNT1 in a head-to-head manner with an interval of less than 7 kb - Wnt10b gene encodes a 389-amino acid protein with 96.6% sequence identity to mouse Wnt10b and it is localized in chr12q13 in human and in chr 15 56.8 in mice |
brain |
gdT-cell |
Non canonical pathway |
||||
Wnt4 |
- Growth factor for Double negative Thymocytes (86) |
- contains 83 absolutely conserved amino acid residues, including 21 cysteines |
ovary, skin, brain and lung |
eosinophil |
Wnt5a |
- Growth factor for mouse fetal liver HSCs, human CD34+ bone marrow cells and human CD34+ cord blood cells. Negative regulator of mouse pre- and pro- B cells and thymocyte development - HSC aging (86- 87-88) |
- 38 to 43-kD cysteine-rich putative glycoproteins, a hydrophobic signal sequence and 21 conserved cysteine residues - Localized in chr3p21.2 in human and in chr14 in mice |
salivary gland, brain, lung, and heart |
Not detected in immune cells |
Wnt5b |
- Expressed by human bone marrow B-cell progenitors (3-83) |
- 359-amino acid protein with an N-terminal signal peptide, 4 N-linked glycosylation sites, and conserved residues of the WNT family. - Shares 80% sequence identity with Wnt5a and it is localized in chr12p13 in human and in chr6 in mice. |
Prostate, fetal brain and at low levels in fetal lung, kidney, adult liver, ovary, and small intestine |
classical monocyte, eosinophil, myeloid DC, intermediate monocyte |
Wnt6 |
- Not identified (86) |
- 365-amino acid peptide containing conserved residues, 2 N-linked glycosylation sites, an N-terminal signal peptide, and an RGD motif and it shares 47.4% sequence identity with WNT1 - contains 4 exons and is clustered with Wnt10a in a head-to-tail manner with an interval of less than 7 kb and it is localized in chr2q35 in human and in chr1 in mice |
brain and at high levels in testis Placenta, adult spleen |
NK-cell, myeloid DC |
Wnt7a |
- Not identified (86-89) |
-349-amino acid polypeptide that has 98% and 64% sequence identity to mouse Wnt7a and human Wnt5a, respectively and it is localized in chr3p25 in human and in chr6 in mice |
Brain, lung, placenta, kidney, testis, uterus, fetal lung, and fetal and adult brain, gallbladder |
memory CD4 T-cell, naive CD4 T-cell |
Wnt7b |
- Produced by macrophages to induce cell death of vascular endothelial cells (81) |
- Localized in chr22q13 in human and in chr15 in mice |
skin |
Low immune cell specificity |
Wnt11 |
- Not identified (84) |
- 354-amino acid protein shared 97%, 85%, and 63% identity with mouse, chicken, and Xenopus Wnt11, respectively. It contains 5 exons and localized in chr11q13 in human and in chr7 in mice |
ureteric buds, the perichondrium, the lung mesenchyme, the urorectal septum, the urogenital folds, the labioscrotal swellings, the cortex of the adrenal gland, adipose tissue, breast |
NK-cell |
Other Wnt signaling |
||||
Wnt2b/Wnt13 |
- Growth factor for human CD34+ bone marrow cells, expressed in human bone marrow B-cell progenitors (3) |
- 372-amino acid polypeptide including a putative secretory signal peptide and localized in chr1p13 in human and in chr3 in mice |
fetal brain, lung, and kidney, retina |
Low immune cell specificity |
Wnt9a |
- Not identified (84-90) |
- Wnt9a shows 75% amino acid identity to chicken Wnt14. 365-amino acid protein containing an N-linked glycosylation site and residues conserved among members of the WNT family and localized in chr1q42 in human and in chr11 32 in mice |
adult skeletal muscle, heart, placenta, adult lung, pancreas, spleen, ovary, fetal brain, and fetal lung |
Not detected in immune cells |
Wnt9b |
- Not identified (90) |
- Wnt9b shows 53% amino acid identity to chicken Wnt14, 54% identity to human Wnt9a, and 65% identity to shark Wnt9. It is localized in chr17q21 in human and in chr11 63 in mice -359-amino acid protein shares significant identity with the 331-amino acid human Wnt9b protein, and both proteins contain all 23 cysteines conserved in Wnt proteins |
ductus deferens, epididymis, seminal vesicle |
Low immune cell specificity |
Wnt16 |
- Target of E2A-PBX in pre-B-ALL; expressed by human bone marrow B-cell progenitors (3) |
-365-amino acid protein with characteristics of a secreted protein, including a hydrophobic signal peptide and many conserved cysteine residues located throughout the protein. It shares 42 to 48% sequence identity with Wnt2b. It is localized in chr7q31 in human and in 6 in mice |
Brain, placenta, spleen, lymph node, appendix, blood, cervix, uterine, ductus deferens, skin |
memory B-cell, naive B-cell |
Table1: The 19 mammalian Wnt proteins, their role in hematopoiesis, their distribution in human body and in the blood.
Phenotypic similarities between Wnt and Porcupine revealed that the latter is dedicated to Wnt signaling and required in Wnt producing cells rather than in transducing Wnt signals. In addition, a study by Hofmann et al., highlighted a sequence similarity between Porcupine and membrane-bound acyltransferases, enzymes that are present in the ER membrane and acylate a variety of substrates, suggesting that Porcupine could encode an enzyme that catalyzes the transfer of palmitate onto Wnt. In accordance with this, it was shown that Wingless, Wnt of Drosophila, loses its hydrophobicity and membrane localization when O-acyltransferase activity is biochemically inhibited or when Porcupine is genetically eliminated. These data show that the main function of Porcupine is Wnt lipidation and membrane targeting [11-12].
After post-translational modification in the ER, palmitoylated Wnt molecules bind to a sorting receptor Wnt ligand secretion (WLS) modulator, which accompanies them to the Golgi. There, V-ATPase-mediated vacuolar acidification allows the release of Wnt molecules from WLS into the extracellular environment where they bind to and activate transmembrane receptors, initiating a variety of Wnt signaling pathways [10] (Figure 1).
Figure 1:: Schematic representation of the major known components of the Wnt pathway with camparison between human, mouse and other models. A- Wnt: the expression and evolution of Wnt among different species showed the conservation of the palmitate group and N-glycosylation sites.
B- Frizzled: Frizzled gene (FRZB) is composed by a total of 6 exons.
C- The predicted structure of wild-type LRP5, the homolog of Arrow in Drosophila: LRP5 contains 4 propeller domains (bleu rectangle) interspersed by four EGF-like domains (green rectangles), three LDL receptor-like ligand binding domains (purple rectangle), a transmembrane domain (black rectangle), and a 200 amino acid residue cytoplasmic domain (blue rectangle). D- A tertiary structure of β-catenin: The β-catenin protein shares 70 % amino acid identity with the product of the Drosphila segment polarity gene "armadillo". The destruction box of β-catenin contains phosphorylation sites for GSK3β and ubiquitination sites. APC, Axin and LEF/TCF binding sites have been mapped to the armadillo repeats of β-catenin.
E- Structure comparison between both APC isoforms in human and Drosophila: APC structure is very conserved among the species and among the two isoforms. It is composed of seven conserved armadillo repeats, a region consisting of 20 amino-acid repeats containing β-catenin binding site and SAMP repeats required for binding Axin. The 15 amino-acid repeats (exists only in human APC2) contains another β-catenin binding site and the Basic Domain (exists only in Drosophila APC2) is a basic microtubule binding region. F- Human and mouse GSK3β: GSK3B is comprised of 12 exons in human and 11 exons in mouse. The ATG start codon is located within exon 1 and the TAG stop codon is found in exon 12 (Human) and 11 (Mouse).
G- Schematic representations of Axin, with its ligands binding to its known structural domains: DIX domain mediates dynamic polymerisation (T. Schwarz-Romond et al., 2007) and RGS domain has been found in proteins that regulate G-protein signaling. H- The domain structures of β-TrCP protein: β-TrCP is homologous to Slimb in Drosophila, which targets Armidillo. There are two isoforms of β-TrCP. β-TrCP1 is paralogous to β-TrCP2. Both of them are called β-TrCP, as their biochemical properties are indistinguishable.
I- DVL structure with conserved DIX sequence alignment among different vertebrate and invertebrate species: DVL homologs are conserved in Drosophila (dishevelled, dsh). DVL contains a DIX domain important for homodimerization, a PDZ domain and a DEP domain required for recruitment of Dishevelled to the plasma membrane. J- Structure comparison between the different isoforms of TCF and among vertebrates and invertebrate species with conserved domains highlighted: TCF is composed of a DNA- binding domain containing a nuclear localization signal (NLS) and a HMG box, which is a DNA binding motif. K- Domain structure of the Dkk family: Human DKK1-4 are shown with their domains DKK_N coressponding to the CRD1 domain (Cys2 domain) in mouse Dkk4, NvDkk1/2/4 and HyDkk1/2/4 and colipase fold coressponding to the CRD2 domain (Cys1 domain) in mouse Dkk4, NvDkk1/2/4 and HyDkk1/2/4. The sgy-domain is only found in human DKK3.
-
Frizzled
Frizzled genes encode seven-transmembrane ligand-activated receptors that bind Wnt proteins. Their amino-acid sequence is composed of a conserved extracellular cysteine rich domain (CRD) at the N-terminal and an intracellular C-terminal domain. Structural studies identified the general hand-like structure of Wnt proteins with thumb and index fingers pinching the extracellular CRD of FZD receptors. On the surface of cells, Wnt proteins bind to FZD through a hydrophobic pocket in the CRD, which recognizes the unsaturated palmitoleic acid of Wnt. Wnt/ FZD binding recruits the single-pass transmembrane molecule LRP5/6, leading to a dimerization and a conformational change of the two receptors. Once phosphorylated by several protein kinases, the cytoplasmic tail of LRP recruits the scaffold protein Axin and the FZD cytoplasmic part can bind to Dishevelled for signal transduction [10].
-
LRP5/6
Wnt signaling requires the presence of a single-pass transmembrane receptor of the LRP family, identified as the gene arrow in Drosophila and as LRP5 or 6 in vertebrates [11]. LRP5 and LRP6 sequences contain 1600 amino-acids and they are 70% identical, and each is 45% identical to Arrow [13]. These two receptors are characterized by the presence of an extracellular domain divided into three segments: β-propeller-EGFs 1 and 2 (P1E1-P2E2), β-propeller-EGFs 3 and 4 (P3E3–P4E4), and the three LDLR type A repeats. Functional-blocking monoclonal antibodies (mAbs) against epitopes in P1E1 and P3E3, respectively, show a distinct inhibition profile toward different Wnt proteins, suggesting a possibility that each β-propeller-EGFs segment have a specific affinity to each Wnt [14]. Indeed, these data infer that Wnt1, Wnt2, Wnt2b, Wnt6, Wnt8a, Wnt9a, Wnt9b, and Wnt10a interact with P1E1, whereas Wnt3 and Wnt3a prefer P3E3. However, a complex composed by Wnt3a–Wnt9b–LRP6 is detected in vitro, suggesting that a single LRP6 and likely LRP5, may engage different Wnts proteins simultaneously [15].
-
β-catenin
𝛽-catenin serves as a platform for a variety of co-activators involved in stem cell maintenance, proliferation and behavior, embryonic development, and adult tissue homeostasis. 𝛽-catenin transcriptional output and regulation are different in cells from different origins such as epithelial cells where high levels of membrane-bound 𝛽-catenin and a large number of adherens junctions were found, while 𝛽-catenin is largely undetectable in normal peripheral blood T-lymphocytes. When the Wnt/𝛽-catenin pathway is acivated, 𝛽-catenin translocates to the nucleus and binds TCF1 and LEF1 nuclear factors to activate transcription [12].
-
Destruction complex
The key event of Wnt Signaling is the release of β-catenin in cells and its stabilization. In the absence of Wnt and in order to keep β-catenin levels low, β-catenin is ubiquitinated and degraded by the proteasome. Four proteins forming the so-called destruction complex were identified: the tumor suppressor proteins Axin1 and/or Axin2, the tumor suppressor protein APC and two constitutively active serine-threonine kinases β-TrCP/Slimb and GSK3β. Briefly, Axin and APC serve as scaffolds causing β-catenin association with GSK3β and its phosphorylation in one or more conserved serine and threonine residues in its amino-terminal region. These phospho-amino acids act as a tag for β-catenin’s interaction with β-TrCP/Slimb and its subsequent ubiquitination. The latter event acts as the next step in β-catenin destruction by the proteasome [12]. We will further evaluate this mechanism by detailed description of each component.
APC: APC was initially identified as a tumor suppressor protein. β-catenin is highly expressed in colorectal adenocarcinoma cell lines harboring mutations in the APC gene. However, the expression of wild-type APC in these cells result in a dramatic reduction of β-catenin levels suggesting that APC is negative regulator of β-catenin stability. Structurally, APC is composed of an N-terminal region required for multimerization; seven armadillo repeats; a region consisting of 15 amino acid repeats that contains a β-catenin binding site; a region consisting of 20 amino acid repeats that contains another β-catenin binding site; SAMP repeats, localized between the 20 amino acid repeats and required for Axin binding; and finally a basic microtubule binding region [12].
GSK3β: GSK3β a serine/threonine kinase that plays a crucial role in regulating β-catenin stability. GSK-3β can phosphorylate one or more highly conserved serine and threonine residues (S33, S37, T41, and S45) in human β-catenin, localized in the `destruction box' sequence, which is required for targeted degradation. A mutation or deletion of these serine and threonine residues could highly stabilize β-catenin [12] and can function as an oncogene in various human tumors.
Some studies showed that a mutation in zeste of white3 (zw3) (GSK-3 Drosophila ortholog) caused the accumulation of Armadillo (β-catenin Drosophila ortholog) and mimic Wnt/β-catenin pathway activation. Other studies highlighted the stabilization and nuclear accumulation of β-catenin following the expression of a GSK3β kinase dead mutant in Xenopus [16,17]. These data constitute a strong evidence for the important role of GSK-3 in regulating β-catenin.
Axin1/2: Axin was first identified as the product of the mouse fused locus (18). The Axin gene is conserved in humans, rats, mice, chickens, Xenopus, and Drosophila. Drosophila possesses only one Axin gene, but vertebrates and nematodes have two, Axin1 which shows 87% similarity to its human homologue and Axin 2/Conductin. However, in the mouse, the only difference between these two isoforms is their patterns of transcription. Axin1 is more widely expressed, whereas Axin 2/Conductin has a more restricted expression pattern that is controlled in part by Wnt signaling [19].
Axin1/2 structure and mechanism are very well conserved. It is a largely unstructured, flexible protein [20] and composed of two conserved domains, an amino-terminal domain RGS referred as Regulator of G-protein Signaling and a carboxy-terminal domain referred as DIX. The RGS domain is required for APC binding [21-23] and DIX for homodimerization [23,24] and for interactions with Protein Phosphatase 2A (PP2A) [24] and Dishevelled [23,24]. However, the central region binds to β-catenin and GSK3β [21,22]. For this reason, it was proposed that Axin could act as a bridge between GSK3β and β-catenin, in order to facilitate GSK3β dependent phosphorylation and the subsequent degradation of β-catenin. Consistent with this proposed role, it was shown that Axin is able to promote GSK3β mediated phosphorylation of β-catenin in vitro. Other data highlighted the low number of Axin molecules comparing to the rest of destruction complex proteins. Therefore, it was suggested that Axin could be a limiting component of the Wnt and could promote the rapid assembly and disassembly of Wnt pathway components to regulate β-catenin stability in cells [11]. It seems that the functional relationship between GSK3β, Axin and β-catenin is reversible. To explain more, β-catenin down regulation mediated by Axin could itself be regulated by GSK3β. As Axin is a substrate of GSK3β, Axin phosphorylation mediated by GSK3β increased its stability in the cell. In the same manner, Willert K et al [25] demonstrated that the state of Axin phosphorylation correlated with changes in Axin ability to bind β-catenin: phosphorylated Axin bind to β-catenin more effectively than the dephosphorylated form.
β-TrCP/Slimb: is a component of SCF ubiquitin ligase complex. The understanding of β-TrCP/Slimb function on Wnt/β-catenin pathway started with some studies showing that the loss of Slimb functions in Drosophila caused a high activation of Wnt/β-catenin pathway and accumulation of high levels of Armadillo [26]. Consistent with this, β-TrCP/Slimb over-expression inhibited Xwnt-8 activity [27] and over-expression of its antagonist stabilizes β-catenin and activates Wnt/β-catenin target genes in Xenopus [27,28]. Based on these data, a model of β-TrCP/Slimb function was designed. Simply, β-TrCP/Slimb binds phosphorylated β-catenin, promoting the ubiquitination of ligase complex and β-catenin and its proteolysis by the 26S proteasome [29].
-
DVL (disheveled)
DVL is a cytosolic adaptor protein involved in canonical and non-canonical Wnt pathway. Wnt activation recruits DVL to the membrane through its DEP domain. During Wnt pathway activation, DVL plays the role of a positive regulator by inhibiting Axin activation. DVL binds to Axin through its C-terminal region and modulates Axin ability to dimerize. Furthermore, DVL, itself could be regulated and phosphorylated following its association with Wnt3 through CKI domain. The phosphorylated form of DVL present a high affinity to Frat and their binding causes GSK3β inhibition. In Wnt stimulated cells, the complex DVL/Frat may prevent GSK3β 3/Axin association and β-catenin phosphorylation [30].
-
TCF/LEF
Almost all vertebrate genome carry a family of four members: T cell factor 1 (TCF7 gene) [31], lymphoid enhancer factor 1 (LEF1 gene) [32], T cell factor 3 (TCF7L1 gene) and T cell factor 4 (TCF7L2 gene). TCF/LEF contains four functional domains: a highly conserved N-terminal β-catenin domain, an HMG DNA binding domain, a context-dependent regulatory domain and an alternatively spliced C-terminal tail. The connection between TCF/LEFs and Wnt signaling is through the β-catenin domain. This binding process involves conformational changes in the first 50 amino-acids. Splice variants lacking the β-catenin domains of TCF/LEF act as dominant negative regulators of Wnt signaling, as their deletion abrogates TCF-mediated transcriptional activation and cause developmental defects in Drosophila and Xenopus embryos [21-31].
During active Wnt/β-catenin signaling, β-catenin is stabilized, accumulated in the cytoplasm and translocated into the nucleus. It can interact with TCF/LEF DNA-binding proteins. Surprisingly, in the absence of β-catenin (Wnt “off” state), TCF acts as a repressor of Wnt target genes by interacting with Groucho proteins. The repressing effect of Groucho is mediated by its interaction to histone deacetylases (HDAC), which are thought to make DNA refractive to transcriptional activation [33]. However, in the Wnt “on” state, β-catenin converts TCF from gene repressor into a transcriptional activator complex. This could be explained by the recruitment of histone acetylase CBP/p300 (cyclic AMP response element-binding protein) and the displacement of Groucho [34].
-
DKK
The most characterized extracellular inhibitors of Wnt signaling are the Dkk proteins. They are expressed in humans and mice but not in invertebrates [35]. Dkk1 binds to LRP5/6 and to another class of transmembrane molecules, the Kremens. This promotes the internalization of LRP5/6, making it unavailable for Wnt reception. In contrast, Dkk2 can act as an activator or as an inhibitor of the Wnt signaling pathway, depending on cellular context and LRP5/6 level. At low level of LRP5/6, Dkk2 functions as a Wnt inhibitor, but, at high level, it acts as an activator. The switch of function is regulated by Kremen2, which invariably turns Dkk2 into a Wnt inhibitor [11].
-
Other receptors
Different Wnt proteins are able to activate distinct intracellular responses in a variety of experimental assays. This response diversity is due to the repertoire of receptors, co-receptor and diverse binding molecules in the cell. β-catenin can be activated by Wnt-independent mechanisms. As an example, expression of Integrin-linked kinase (ILK) in mammalian cells phosphorylates and down-regulates GSK3β activity [36] and thus, promotes β-catenin nuclear accumulation and stabilization. In addition, it was shown that mutated presenilin causes the decrease of β-catenin [37].
Besides Wnt, FZD receptor can interact with cysteine-knot protein Norrin encoded by the NDP gene. In human, NDP mutations cause Norrie disease characterized by hypo vascularization of the retina and a severe loss of visual function. Norrin has no sequence similarity with Wnt, but, express a high affinity to FZD-4 [37]. Once associated to FZD-4, LRP5 binds to the complex FZD-4/Norrin and leads to the potential activation of Wnt/β-catenin pathway. Furthermore, it was demonstrated that Tspan12 could be a Norrin-specific co-receptor that could be involved in Wnt/β-catenin pathway by forming a ternary complex Tspan12/ Norrin/FZD-4 [11-37]. In addition to the core receptors FZD and LRP5/6, there are several other transmembrane molecules implicated in Wnt signaling including ROR and RYK tyrosine kinase receptors. Dwnt-5, RYK transmembrane tyrosine kinase and a regulator of axon guidance in the Drosophila central nervous system (CNS) and embryos, could bind to Wnt via its Wnt-interacting WIF domain and activates Wnt down-stream target genes [11]. Nonetheless, ROR tyrosine Kinase receptor binds to Wnt via its CRD domain. Upon its activation, it binds with DVL, leading to Wnt pathway component phosphorylation [12].
Different receptor and co-receptor interacting with Wnt and FZD are involved in Wnt pathway. Therefore, it will be very interesting to understand whether these different receptors function in the same tissues and cellular processes and whether Wnts simultaneously contact FZD/LRP and the rest of the receptor and co-receptors or in parallel pathways.
Different Pathways
-
β-catenin Wnt signaling
The canonical β-catenin pathway is the most studied of the Wnt-signaling cascades. It is shown to be crucial in numerous developmental processes including embryogenesis and remains essential throughout life given its essential role in stem cell self-renewal, in adult stem and progenitor cells. The hallmark of this pathway is the cytoplasmic accumulation of β-catenin, its stabilization and its nuclear translocation and activity. The canonical β-catenin signaling is initiated by Wnt proteins binding to one of FZD receptors and co-receptors LRP5/6 on the cell surface. This binding triggers the activation of DVL and its recruitment to the intracellular C-terminal domain of FZD. The latter interacts with Axin and provokes GSK3β dissociation. Therefore, GSK3𝛽 is inactivated and prevented from phosphorylating 𝛽-catenin which is spared from degradation. As a consequence, 𝛽-catenin accumulates in the cytoplasm and eventually is translocated to the nucleus. In this case, it binds DNA through its association with TCF-1 and LEF-1 in order to regulate the expression of specific target genes [1-10] (Figure 2).
Figure 2: Wnt signaling pathway. A- Wnt off. In the absence of Wnt proteins, β-catenin is phosphorylated and degraded due to the presence of the destruction complex and TCF/LEF proteins are in repressed state and bound to Groucho co-repressor. B- Wnt/β-catenin pathway. In the presence of Wnt, Wnt binding to FZD receptor and its co-receptor LRP5/6 leads to the dissociation of the destruction complex and to β-catenin accumulation and its nuclear translocation. This leads to the transcription of target genes. C- Planar polarity pathway. Wnt binding to FZD receptors promotes DVL recruitment which triggers multiple β-catenin independent non-canonical downstream events to mediate cell migration, motility and polarity. D- Ca2+ pathway. Activation of G proteins and phospholipases leads to the increase of Ca2+ cytoplasmic level and the activation of PKC, CAMKII and the phosphatase calcineurin. This leads to NFAT translocation to the nucleus and the expression of target genes. E- YAP-TAZ pathway. Wnt binding to FZD insures the activation of G-proteins Gα12 and Gα13 leading to the translocation of YAP-TAZ to the nucleus and the activation of target genes. F- STAT3 pathway. Wnt5A binding to Frizzled-2 activates Src family kinases Fyn and STAT3. STAT3 migrates to the nucleus and trigger STAT3-mediated EMT processes. This non-canonical pathway is negatively regulated by Abl interactor 1 (ABI1). ABI1 can directly interact with either Fyn or STAT3. Abbreviation: TCF= T-cell factor; LEF= lymphoid enhancer factor, TLE= transducin-like enhancer of split; LGS=legless; HDAC=histone deacetylase, PKC= protein kinase C; CamKII=calmodulin kinase II. NFAT= Nuclear Factor of Activated T cells; PLC= Phospholipase C; IP3= inositol tri phosphate, AP1= activator protein 1; CDC42=cell-division cycle 42; DAAM= Dishevelled-associated activator of morphogenesis; NFAT= nuclear factor of activated T cells; PDE6=phosphodiesterase.
-
Non canonical pathways
Non-canonical Wnt pathways or β-catenin independent pathways, as their names imply, are completely independent from β-catenin/TCF/LEF binding in order to modulate downstream signaling. Some of these pathways directly regulate cellular actin dynamics and modulate actin-related events such as polarity, migration, and adhesion and other non-canonical pathways elicit transcriptional responses [1-10]. Currently, five non-canonical Wnt pathways have been identified:
Wnt/Ca2+ pathway: As the first non-canonical pathway, Wnt Ca2+ pathway is initiated by Wnt binding to FZD receptors and DVL recruitment, but in this case, to activate heterotrimeric G proteins. Once activated, G proteins trigger phosphatidylinositol signaling in order to stimulate the release of Ca2+ and the activation of Ca2+-dependent effector molecules. Several Ca2+-sensitive targets—protein kinase C, Ca2+– calmodulin-dependent protein kinase II (CamKII), and the Ca2+-calmodulin–sensitive protein phosphatase calcineurin—have been identified as Wnt- Ca2+ pathway targets in vertebrates. The increase of Ca2+ intercellular level and the activation of protein kinase C and Ca2+/ calmodulin-dependent kinase stimulateTAK1 mitogen-activated protein kinase (MAPK) and NLK MAPK. These protein kinases phosphorylate TCF/LEF factors and inhibit the interaction of β-catenin-TCF/LEF complex with DNA [2].
Wnt/PCP pathway: Planar cell polarity (PCP) is manifested in Drosophila wing, eye, and sensory-bristle development. The PCP pathway shares two key components of the canonical pathway: FZD and DVL, but different downstream components likely including RhoA, RhoA-associated kinase (ROCK), Rac, and JNK. PCP pathway is initiated by Wnt binding FZD/LRP5/6 complex. Then, CK1ε associates to LRP5/6 through E-cadherin/p120-catenin and interacts with PR61ε, a subunit of PP2A phosphatase that controls its activity. This promotes CK1ε C-terminal tail dephosphorylation and its activation. However, in some cases, Wnt could promote CK1ε activation by its binding to RNA helicase DDX3 or to the non-canonical Wnt co-receptor ROR2. In any way, activated CK1ε recruits DVL2 to FZD/LRP5/6 complex, allowing the formation of protein aggregate known as signalosome. DVL2 can bind and activate phosphatidylinositol 4-kinase IIα and phosphatidylinositol 4-phosphate 5-kinase 1β, generating PtdIns(4,5)P2 and favoring CK1γ association to LRP5/6 through AMER/WTX. This is the diverging point of the two signaling pathways, as CK1γ phosphorylates LRP5/6 in Thr1479, creating a docking site for Axin and enhancing GSK3-β interaction with LRP5/6 complex. Axin association with LRP5/6 causes GSK-3β inhibition and precludes β-catenin phosphorylation at Ser37, its ubiquitination and degradation. However, the mechanism behind GSK3β inhibition is still not elucidated. Several controversial models have been proposed, but further investigations are required.
Negative regulatory mechanisms of the Wnt/PCP pathway are still not well understood. According to some studies, the signalosome could be enhanced by TMEM59, as it interacts with FZD and LRP5/6 and so blocks DVL2 association with FZD/LRP5/6. Other studies suggested tetraspanin-like transmembrane scaffold protein Van Gogh (Vangl in mammals) and intracellular scaffold Prickle (Pk) as an antagonist signal to Wnt/PCP patway. Vangl and Pk form a complex and recruit proteins that negatively regulate the Wnt/PCP pathway either by mediating DVL ubiquitination and proteasome degradation, or directly by altering Rho GTPase-mediated actin cytoskeleton remodeling and JNK-mediated transcriptional responses [30].
Wnt/ROR pathway: Receptor tyrosine kinase-like orphan receptors (RORs) contain an extracellular Cystein Rich Domain (CRD) for Wnt recognition and an intracellular tyrosine kinase domain. There are two well-known RORs: ROR1 and ROR2. ROR2 could play the role of a co-receptor for FZD receptors to trigger non-canonical Wnt signaling. ROR2/FZD complex promotes serine/threonine phosphorylation of the ROR2 intracellular tail, the recruitment of downstream scaffold proteins and the initiation of signal transduction. At the same time, ROR2 itself can also serve as an individual receptor for Wnt molecules to stimulate multiple non-canonical signal events, like actin remodeling and JNK-mediated transcriptional responses [10].
Wnt/Yap–TAZ pathway: YAP-TAZ can be activated following the negative regulation of Hippo pathway modulators larger tumor suppressor 1 and 2 (LATS1/2) by Wnt. Usually, LATS1/2 promotes YAP–TAZ complex phosphorylation, its ubiquitination and degradation. However, Wnt–Fzd–ROR complex stimulates Ga12/13 activation and Rho’s, which inhibits LATS1/2 and stabilizes the YAP–TAZ complex. This will lead to the activation of downstream transcriptional responses [10].
Wnt–FYN–STAT3 pathway: This pathway is initiated by Wnt5a/b binding to FZD2 receptor and its tyrosine phosphorylation on the C-terminal. In this scenario, Src family kinase FYN will be recruited through protein–protein interactions and activated, and thus tyrosine phosphorylation of STAT3 will be promoted [10].
Feedback Loops
Wnt signaling pathways are strictly regulated in developmental and oncogenic processes. One of the mechanisms of regulation is its direct modulation by oxidization and abrogation through a transmembrane protease, Tiki-mediated N-terminal cleavage. In addition, Wnt deacylase Notum removes the palmitoleic acid on Wnt molecules, modifies them extracellularly and causes the loss of binding to their ligand.
In addition to the above-mentioned negative regulation of Wnt proteins, several negative-feedback mechanisms exist to abrogate Wnt signaling at the receptor and co-receptor level. The most important target is FZD receptor. In Drosophila, following its activation, Wg down-regulates DFZ2, in order to limit Wnt signaling and to allow Wg diffusion over longer distance (39). In addition, Dsh, a ubiquitously expressed cytoplasmic protein, directly binds to FZD through its C-terminal cytoplasmic Lys-Thr-X-X-X-Trp to transduce Wnt signal into the cell (40). Surprisingly, β-catenin signaling induces the up-regulation of transmembrane E3-ubiquitin-ligase ring finger protein 43 (RNF43) and zinc-ring finger 3 (ZNRF3). The latter promotes FZD receptors ubiquitination at the plasma membrane, leading to FZD receptor endocytosis and its proteasome degradation. Furthermore, Wnt molecules could interact with other secreted proteins that contain CRD such as Wnt inhibitory factor and secreted Frizzled related proteins (sFRPs), which prevent them from binding to FZD receptors [11].
Similar to FZD, LRP may also interact with a cytoplasmic component of Wnt-signaling pathway. It is known that LRP binds to FZD in order to stabilize β-catenin, but, in case of down regulation, LRP binding to FZD could be blocked by Wnt antagonist. The most cited antagonist is Dkk1 which form a ternary complex with Kremen and LRP5/6. This ternary complex is rapidly endocytosed to stop Wnt signaling cascades [11].
Other studies showed that the Wnt pathway can be regulated at the level of downstream signal transduction genes in the nucleus such as TCF. TCF/ β-catenin/CBP complex could interfere with Brg-1, a component of the SWI/SNF (switching-defective and sucrose non-fermenting) complex and generate chromatin remodeling that favors target gene transcription [41]. In the same manner, chromatin remodeling could be mediated by Legless (Bcl9) and Pygopos, two genes that promote Wnt signaling in mammalian cell culture experiments [42]. In the nucleus, Wnt signaling is under the control of different proteins that could target TCF. For example, β-catenin-binding protein, ICAT blocks β-catenin binding to TCF [43] and leads to β-catenin, LEF, and CBP/p300 complexe dissociation [44]. Also, in some cases, TCF can be phosphorylated via MAP NLK/Nemo [45]. As a result of this phosphorylation, DNA-binding affinity to β-catenin/TCF/LEF complex is reduced and transcriptional regulation of Wnt target genes is affected [45,46].
An important positive-feedback mechanism has been revealed to amplify Wnt signaling in early embryonic development, stem cell renewal, and tissue homeostasis. This feedback is carried by R-spondins, identified as Wnt agonist. They don’t possess intrinsic signaling activity, but they are able to interact, in a high affinity manner to Lg4, Lg5 and Lg6, 7-TM receptors, which are essential for enhancing low doses of Wnt [47]. The Lgr binds to R-spondins through its Furin-2 repeat, allowing its interaction with RNF43/ZNRF3 and the formation of a ternary complex. This complex leads to membrane clearance of the E3 ligases and to the decrease of RNF43/ZNRF3 levels in the plasma membrane preserving FZD receptors to amplify both Wnt/β-catenin pathways [11,12].
Transport of Wnt proteins between cells
Wnt can act on distant neighbors in a concentration dependent manner [39], but, the questions here are whether palmitoylated Wnt molecules are actively transported, how they are released from cells, and move over long distances. There is no evidence for a specific exporter of Wnt molecules, but, few export models have been proposed. F. A. Ramirez-Weber and T.B. Kornberg [48] came up with the idea that in Drosophila, chicken, frog and fish, Wnt can be transported by thin filopodial processes called cytonemes. In addition V.Greco et al., [49] suggested that, in Drosophila wing imaginal discs; Wnt is transported as cargo outside the cells via a vesicle-based transport, called argosomes. Other studies highlighted a further transport mechanism on exosomes. Recent data indicated that apically released Wnt3a and Wnt11 can be found on CD63-positive exosomes, whereas basolateral secreted Wnt3a is co-fractioning with TSG101 exosomes. A possible explanation for this process is that Wnt ligands are first presented at the apical membrane to mediate short-range signalling, before being re-endocytosed, packaged into endosomes and transported to the basolateral membrane for secretion [50]. The secretion mechanism of exocytosis requires the passage of secretory cargo from endosomes into multivesicular bodies (MVBs). Another study realized in Drosophila highlighted the presence of HSPG, a gene that can act as a co-receptor on target cells, stabilize Wnt or help in its presentation or movement between cells [11].
Roles of Wnt during hematopoiesis and lymphopoiesis in human and mice
We here discus the relatively limited number of studies focusing on normal Wnt signaling in human cells, in reference to the studies done in mice and the above described properties of the Wnt pathway.
-
Hematopoietic Stem cells (HSCs)
Wnts exert a wide variety of effects on target cells during development. The most important involve theirrole in healthy stem cells, characterized by their self-renewal ability and their ability to generate cells with functional diversity (Figure 3), and they have a role in maintaining and inducing an undifferentiated phenotype in hematopoietic cells. A study realized on Wnt3a-/- mice showed an important decrease of the lineage-negative, Sca1+, Kit+ murine bone marrow (LSK) population, which contains murine HSCs [51]. These Wnt3a -/- HSCs were devoid of self-renewal, as demonstrated by lack of reconstitution in secondary recipient mice. It was subsequently shown that the Wnt3a deficiency leads to a complete loss of canonical Wnt signaling in HSCs, explaining in part the dramatic phenotype of losing self-renewal [52].
In vitro experiments on human HSC and HPC (hematopoietic progenitor cells) revealed crucial roles of Wnt in multilineage reconstitution and differentiation into more committed cells. High dosesages of Wnt3a completely blocked the differentiation of CD34+CD38− HSCs into CD19+ B-lineage lymphocytes and into natural killer and plasmacytoid dendritic cells (53). It is known that CD34+CD38− HSCs or CD34+CD38+ hematopoietic progenitor cells (HPCs) express c-Kit and its expression decreases during development. However, in the latter study, the expression of c-Kit remained high in the presence of Wnt3a [53]. This blockade is explained by the fact that Wnt signaling could be required during a narrow time window for human hematopoietic development (in vitro is approximately between day two and day four of differentiation), as Wnt signaling inhibition from day 2 to day 4 significantly decreased the differentiation efficiency of CD34+HPC and it increased their differentiation during days 2–4 of the culture, while during other time windows, it had no effect [54].
Besides Wnt, HSC express various Notch receptors. Based on the gain and loss of function studies that demonstrated the crucial role of Notch in regulating T versus B cell lineage decisions, a hypothesis about Notch and Wnt connection during multilineage reconstitution and differentiation into T-cells has been established. In the presence of Wnt3a and Delta1ext-IgG (an activator of Notch), CD34+CD38- HPC maintained the expression of CD34, but in the same time, they adopted an early lymphoid progenitor phenotype (CD34-CD7+CD445RA+). In addition, Wnt and Notch can cooperate in early T cell development, as together; they induced the expression of both CD3ε and pre T α [5].
The role of Wnt signaling in human HSCs and primitive lymphoid progenitors is controversial. Wnt3a was able to differentiate Lin−CD34+CD38−CD7+ early lymphoid progenitors (ELPs) into lymphoid lineage cells and also generated GPA+CD14−CD19− erythroid cells, but no effect on Lin−CD34+CD38+CD10+ common lymphoid progenitors (CLPs) was observed [53]. In the same manner, another study showed that Wnt3a accelerated the decline of UCB-derived CD34+ cells frequency, leading to a significant reduction of their expansion. This effect has its greatest impact on multi-lineage CFUGEMM (granulocyte, erythrocyte, monocyte, megakaryocyte) and has no impact on more committed population such as BFU-E (Burst Forming Unit-Erythrocyte) and CFU-GM (granulocyte, macrophage). The reduction in frequency of most immature CFU and the higher frequency of lineage positive cells suggest that Wnt3a promotes rather than inhibits growth factor-driven differentiation of CD34+ cells in expansion cultures. However, this effect is contradictory to its long-term repopulation ability, as a very low level of human chimerism was observed in the bone marrow of lethally irradiated recipient mice transplanted with Wnt3a treated CD34+cells (55). We interpret these results in the context of the importance of Wnt dosage, which was shown in mouse studies [56-58] (Figure 3).
Figure 3: Hematopoietic stem cell development.
-
B-cell development
Several studies have indicated that canonical Wnt signaling has a pronounced role in B-cell progenitors in the bone marrow, while more mature stages do not depend on Wnt. Wnt3a deficient mice have a severe reduction of myeloid, granulocyte and monocytes frequencies in fetal lever, but, CLP and precursor B are not affected. Unlike B-1 precursors, the measure of Axin expression within B-1 population showed the complete inactivation of Wnt signaling. The co-culture of B-1 precursor cells with OP-9 Wnt3a indicated that Wnt3a promoted their differentiation into B-1a cells [59]. Differentiation patterns were quite different during the co-culture of B-1 precursor cells with OP9-Wnt5a where a potential increase in their number with the loss of the AA4.1 expression were observed, suggesting that Wnt 5a induced the proliferation and differentiation of B-1 precursors into B-1 cells [59]. Taken together, these findings lead to the two following hypotheses: 1- Wnt3a and Wnt5a have complementary effects during B lymphopoiesis: Wnt3a is involved in pre-pro B differentiation, then, Wnt5a promotes B-1 differentiation to a more committed population. 2- Wnt3a was shown to negatively regulate early B cell proliferation possibly by inhibiting a Wnt5a effect, and Wnt5a was shown to down- regulate B-1 cell proliferation probably by canonical Wnt target gene inhibition. In any case, these two hypotheses need more verification and investigation in order to better understand the functional relationship between Wnt5a and Wnt3a in B lymphopoiesis.
In human bone marrow, the mRNA level of membrane receptor LRP5, LRP6, Fzd5 and Fz6 decreased as the cells developed from small pre B into immature B cells. Indeed, Wnt3a inhibited the development of BM HSC (CD133+CD10-) into more committed lineage and caused a severe reduction of CD19+ cells. Similarly, culturing CD10+ B progenitor cells in Wnt3a conditioned medium led to a very significant decrease of CD19+ total number. In addition, Wnt3a has an inhibitory effect on CD34+ cells (pro-B) and CD20+ (pre-B). This led to the conclusion that Wnt3a has an important inhibitory effect, not on the relative proportions of different bone marrow B subpopulations, but on pro B, pre B and immature B cells. This inhibitory effect is due to the inhibition of pro B and pre-B cells proliferation in a dose dependent manner [3]. It seems that the Wnt canonical pathway is conserved, as it has a similar effect on human and mouse bone marrow progenitor cells.
-
T-cell development and maturation
In both humans and mice, T cell development proceeds through a series of very regulated stages, characterized by the expression or absence of specific membrane molecules: DN (double negative: CD4-CD8-), DP (double positive: CD4+CD8+), SP(single positive: CD8+CD3+ or CD4+ CD3+). The DN subset can be further subdivided into four stages in mice and into 3 previously defined stages in humans [60].
Early T cell: Several lines of evidence indicate that Wnt signaling has an important effect on DN stages and during DN to DP transition. It was shown that Wnt3a acts in a dose dependent-manner, such that Wnt3a over-expression blocked T cell development at DN1 stage [61]. Interestingly, decreasing the Wnt3a level promoted the development of thymocytes into DN3 and DN4, but, its genetic abrogation severely impacted DN2 and DN3/4 proliferation [51]. In line with this, pharmacological inhibition of Wnt by ICAT abrogated the transition of CD4-CD8- DN to CD4+CD8+ DP, without affecting later stages of development (60).This inhibition was also shown to affect human T cell development in fetal thymic organ cultures (FTOC) in which human T cell can develop fully in the context of E14 murine embryonic thymi. In these experiments, DKK1 could inhibit early development of thymocytes. Human thymocytes undergo Wnt signaling as measured by LacZ based reporter constructs and express Fz6 at high levels [62].
CD8+ T cell: important work by Gattinoni and colleagues [64] indicates that canonical Wnt signaling induced by activated β-catenin inhibited CD8+ maturation into T effector cells. Active β-catenin decreased the T cell specific killing ability and preserved the release of IL-2 and the naïve phenotype CD44low CD62Lhigh. Gene analysis showed that CD44low CD62Lhigh population expressed, in a low level, the core phenotypic traits of T memory stem cells such as Sca-1, CD122 and BCL-22, indicating that Wnt signaling generated a novel CD8+ T memory population called T memory stem cell. This novel population possesses high self-renewal ability and generated T memory cell, T effector memory and T effector cells in serial transplant experiments (63). Consistently, active β-catenin in naïve CD8+ T cells co-cultured with Wnt3a over-expressing mDCs induced an important proliferation of CD44+CD62L- T memory [64].
Treg: Regulatory T (Treg) cells are of critical importance for maintaining immune homeostasis and tolerance through a variety of incompletely understood cellular mechanisms. The transcription factor FOXP3 is crucial for both Treg cell function and development. β-catenin accumulation in human T-reg promoted TCF activation, which significantly increased Il-2 promoter activity and its protein concentration. This led to the total abrogation of the suppressive capacity of T-reg in vivo [65]. Other studies in mice provide evidence that the suppressive function of T reg cells could be reproducibly and substantially increased in case of abrogation of TCF1 activity or in case of T cell treatment with Wnt inhibitors [65]. The reason for this species difference is unclear and warrants further investigation in order to understand whether the Wnt canonical pathway acts in a dose dependent-manner, whether in a cell line dependent manner, in species dependent manner or on these three conditions at the same time.
-
Natural killer and DC
The first reports of the importance of Wnt signaling in DC arose from studies indicating that the activation of β-catenin/TCF pathway in DCs by Wnt3a, Wnt5b and Wnt16 and the non-canonical pathway by Wnt5a Wnts can condition DCs to a regulatory state [66-68]. In vitro DC exposure to Wnt3a and Wnt5a caused the secretion of a high level of regulatory factors such as retinoic acid (RA), IL-27 and VEGF and a low level of inflammatory cytokines such as IL-6, IL-12 and TNF-α in response to various TLR ligands, suggesting that Wnt3a and Wnt5a conditioned DC to exhibit regulatory functions [67-69]. In accordance with these findings, Wnt-conditioned DCs promote regulatory T cell responses and can suppress disease in experimental models of multiple sclerosis [67]. However, both Wnt3a and Wnt5a had no effect on MHCII and costimulatory molecule induction, and only minimally reduced CCR7 upregulation, and they upregulate immune regulatory cytokines without inhibiting DC maturation, allowing development of a mature tolerogenic DC phenotype [67-69]. It seems that both Wnts induced tolerogenic changes in DC, but the responses they produced were distinct. In studies of primary or in vivo Flt3L-expanded lymphoid tissue-derived DC both Wnts stimulated immune regulatory cytokines, but Wnt3A preferentially induced TGFβ while Wnt5A was a potent stimulus for IL-10 production [69].
Besides the role in the development of a mature tolerogenic DC phenotype, Wnt signaling has been also implicated in early stages of myeloid cell differentiation. Wnt3a increased the proportion of CD11c+ DC expressing MHC II or CD86. Contrary to Wnt3a, Wnt5a decreased the differentiation of DC manifested by the decrease of CD11c+. Consistently, Lin−c-kit+Sca-1+CD34− HSC or Lin−c-kit+Sca-1−CD34+ CMP in Wnt3a conditioning myeloid long-term culture medium promoted a fully mature DC, whereas, Wnt5a completely inhibited it. The same effect was observed with later stages of differentiation. Wnt3a treatment of Gr-1+CD11b+ resulted in a significant increase in DC proportion. To conclude, in early and later stage of myeloid cell differentiation, Wnt3a antagonizes the non-canonical Wnt signaling [69].
Despite the few differences in DC differentiation between humans and mice, the Wnt pathway has similar effect in both species. In both mice and humans, Wnt pathway activation promotes DC differentiation, whereas its inhibition abrogates DC differentiation. For instance, in the presence of Wnt activator SB216763, human CD34+ progenitor cells were able to generate Lin- HLA-DR+ DCs, whereas, Wnt inhibitory factor-1 Wif-1 decreased the proportion of DCs. Cells generated in the presence of Wif-1 had substantially reduced ability to stimulate allogeneic T cells. In contrast, cells generated in the presence of SB216763 had a significantly greater ability to stimulate allogeneic T cells, confirming that Wnt pathway activation leads to the increase of human DC differentiation [69].
In addition to its role in DC differentiation, Wnt3a appeared to play a role in Natural killer cell differentiation. The co-culture of Wnt3a pretreated CD34+CD1a- cells in the presence of Wnt3a, SCF and IL-15 with thymic lobes derived from 15 days old embryos (FTOC) showed an increase of the NK population [68]. This population was able to generate the expression of CD94 and NKG2D, suggesting that an earlier acquisition of these receptors could be a result of transient stabilization of β-catenin. However, the co-culture of Wnt3a pretreated CD34+CD1a- cells with the ST2 cell line in the presence of IL-7 and Flt31 generates a population of CD14+ monocytes is generated, and within the CD14- population, the expression of CD1a, CD11c and HLA-DR were detected, indicating the presence of cDC [68].Taken all these results together, progenitors could differentiate into NK or into monocytes, depending on culture microenvironment and the cytokines. Thereby, understanding the mechanism, the microenvironment and conditions that lead progenitor cells to develop into a specific lineage is an interesting, understudied topic
-
Aging of mature cells
The development and function of DC populations are altered during the process of aging. However, the molecular mechanisms responsible for these changes in aged mice have not been thoroughly investigated. A study by J Xiao et al., [4] reported that the differentiation of both plasmacytoid dendritic cells (pDCs) and CD8α+ conventional dendritic cells (cDCs) are deficient in aged mice, whereas Wnt5a, a non canonical Wnt, is markedly increased in the hematopoietic precursors. Wnt5a treatment of LSK cells caused a significant decrease in the number of Flt3+ LMMP, suggesting that Wnt5a negatively regulated DC development through the impairment of LSK cell differentiation. This was occurred by down-regulating the expression of mRNA genes though to be important for DC homeostasis such as Gifi-1, Ikaros, Bcl11 and Il-7. The decrease of Ikaros and Flt3 in aged LSK diminished DC subsets differentiation in aged mice. Interestingly, pharmacological inhibition of Wnt5a through CASIN treatment of aged LSK cells rejuvenated aged DC differentiation by increasing the proportion of PDCs to a similar level in young LSK cells [4].
CD8+ T-Stem cell self-renewal is a vital feature of immune memory T cells that preserves the lifetime health of an individual. This requires the preservation of T-cell stemness and the flexibility of various T-cell compartments to alternate between a persistent quiescent state and a proliferative state where specific clones divide asymmetrically to give rise to activated memory and T effectors cells, which are vital for pathogen clearance during antigen re-exposure. Connected with these observations, a hypothesis that chronological human-aging would affect T-CD4 stem cell memory (TSCM) renewal capacity was established. It was shown that TSCM and CD31+ naïve frequencies decreased in an age dependent manner. Several clusters of TSCM exhibiting differential expression of OTK7, CD31, CD127, CD150 and CXCR4 were identified in young donors with high expression and in old donors in low level. Surprisingly, gene analysis of different clusters TSCM revealed the presence of a very low level of canonical Wnt key targets such as CTNNB1, FZD4, RAC1 and a high level of pro-inflammatory ligands such as IFNγ. Thus, it is possible that TSCM homeostasis deregulation could be the result of Wnt signaling alteration through the high level of inflammation signatures and could lead to the loss of their proliferative capacity. In accordance with this, young CD4 T cells showed a high proliferative ability compared to old CD4 [70-71].
TSW119 (inhibitor of GSK-3β) treatment of aged TSCM restores their young phenotype and recovers CD127 expression. This population expressed lower level of activation markers: CD95, CD26, CCR5 and CXCR3 and have greater proliferative, functional and trafficking potential. Thereby, the modulation of the Wnt/β-catenin pathway with high concentration of agonist may be helpful in delaying or preventing the loss of memory T cell precursor and naïve T cell homogeneity, which could alter the immune response to new or repeated infections [72]. Taken all these observation in humans and mice together, the pharmacological inhibition of the non-canonical pathway could be a new strategy for the treatment of DCs and T cells in aging related immune-disorders.
-
Wnt signaling in thymus
The thymus, a primary lymphoid organ, provides a unique microenvironment for the development and maturation of naïve T cells. Thymic epithelial cells (TEC) are essential for the attraction of lymphoid precursors into the thymus and their subsequent differentiation, proliferation and selection [74]. Wnt receptor and Wnt4 are widely and exclusively expressed on TEC. The absence of Wnt4 suppresses fetal and postanal thymic expansion and leads to the decrease of TEC number (74). Similarly, the secreted Wnt inhibitor DKK1 developed features similar to thymic aging such as thymic atrophy, TEC reduction and the decrease of their proliferation [74-75]. However, with ageing, many canonical Wnts, including Wnt4 and its downstream gene Foxn1 are downregulated [76].
The inhibition of Wnt signaling in human thymus results in all three hallmark processes of thymic involution. It arrest thymocyte differentiation, and causes the loss of TEC corticomedular organization, and induces epithelial derived differentiation of adipocytes. In healthy thymus, mesodermal cells avoid entering an adipogenic program by secreting Wnt proteins. The decrease of Wnt signaling and its disruption result in the increase of PPARγ expression and spontaneous adipogenesis. Several reports indicated a link between thymus ageing and the increase of PPARγ, which could be also associated with the decrease of peripheral naïve T cell numbers and the decrease of TCR diversity. These finding indicated that Wnt pathway inhibition could be a possible mechanism of human thymic involution and the modulation of Wnt inhibitor secretion could be an interesting target for reversing the atrophy process and boosting adulthood thymic function [77].
Conclusion
Decades ago, Wnt ligands were identified as a growth factor for stem cells. Since then, studies focusing on Wnt signaling have elucidated their complex role in different developmental stages. This review summarizes signaling pathways and the best kown receptors, co-receptors and ligands of this pathway. Given the number of Wnt down-stream genes and their widely ranging functions, but also the limited knowledge of Wnt signaling in human hematopoiesis, we have reviewed the intriguing role of Wnt in the human immune system while comparing it with Wnt signaling in murine cells. Analysis of published datasets shows controversial results about Wnts’ effects on cell behavior, differentiation and proliferation depending on the species. This is in accordance with observations that effects in human and murine models sometimes differ and this may cause confusion regarding real effects in human cells. Direct extrapolation of results from mice is not always possible or desired and underscores the need to conduct more experimental studies with human cells [78-82].
Several authors reported that manipulation of canonical Wnt signaling affects expansion and differentiation potential of HSCs. It is important to mention that the timing of exposure, concentration of ligand and its biochemical origin (artificial stimulation, authentic (recombinant) Wnt stimulation) is a determinant to reflect the effect of Wnt pathway on cell behavior. So, maybe the challenge and focus of future studies will be determining the right concentrations and microenvironment that lead to the differentiation into a specific mature lineage. In this review, we pointed out that the Wnt transcriptional response requires the employment of multiple proteins for β-catenin translocation to the nucleus and the possible crosstalk among pathways [82-91]. Several years ago it seemed next to impossible to conduct functional experiments with human cells to study Wnt signaling. However, the advent of immune deficient mice that allow efficient transplantation of human cells and their differentiation [78], as well as the means to conduct reporter assays and loss of function mutants using CRSPR-Cas9, makes this a distinct possibility in the near future.
Acknowledgements
Dr. Jridi is supported by a Leading Fellows Stipend from a collaborative grant by Leiden Medical School, Leiden University, Delft University of Technology and Erasmus Medical Center. This project has received funding from the European Union's Horizon 2020 research and innovation programme under grant agreement No. 755170.
References
- Pereira CP, Bachli EB, Schoedon G. (2009) The wnt pathway: a macrophage effector molecule that triggers inflammation. Curr Atheroscler Rep. 11(3):236-42.
- Jia L, Miao C, Cao Y, Duan EK. (2008) Effects of Wnt proteins on cell proliferation and apoptosis in HEK293 cells. Cell Biol Int. 32(7):807-13.
- Døsen G , Ellen T, Marit Kveine N, Heidi S, Steinar F , et al. (2006) Wnt expression and canonical Wnt signaling in human bone marrow B lymphopoiesis. BMC Immunology. 7:13.
- Xiao J, Zhou H, Wu N, Wu L. (2015) The non-canonical Wnt pathway negatively regulates dendritic cell differentiation by inhibiting the expansion of Flt3(+) lymphocyte-primed multipotent precursors. Cell Mol Immunol. 13(5):593-604.
- Aoyama K, Delaney C, Varnum-Finney B, Kohn A D, Moon RT, et al. (2007) The interaction of the Wnt and Notch pathways modulates natural killer versus T cell differentiation. Stem Cells. 25(10):2488-97.
- Luis TC, Weerkamp F, Naber BA, Baert MR, de Haas EF, et al. (2008) Wnt3a deficiency irreversibly impairs hematopoietic stem cell self-renewal and leads to defects in progenitor cell differentiation. Blood. 113(3):546-54.
- Blauwkamp TA, Nigam S, Ardehali R, Weissman IL, Nusse R. (2012) Endogenous Wnt signalling in human embryonic stem cells generates an equilibrium of distinct lineage-specified progenitors. Nat Commun. 3:1070.
- Huggins IJ, Bos T, Gaylord O, Jessen C, Lonquich B, et al. (2017) The WNT target SP5 negatively regulates WNT transcriptional programs in human pluripotent stem cells. Nat Commun. 8(1):1034.
- Vijayaragavan K, Szabo E, Bossé M, Ramos-Mejia V, Moon R, et al. (2009) Non-canonical Wnt signaling orchestrates early developmental events towards mesodermal hematopoietic cell fate from human embryonic stem cells. Cell Stem Cell. 4(3):248-62.
- Xiang L, Maria AO, Leszek K. (2020) The physiological role of Wnt pathway in normal development and cancer. Exp Biol Med (Maywood). 245(5):411-26.
- Logan CY, Nusse R. (2004) The Wnt signaling pathway in development and disease. Annu Rev Cell Dev Biol. 20:781-810.
- Nusse R, Clevers H. (2017) Wnt/β-Catenin Signaling, Disease, and Emerging Therapeutic Modalities. Cell. 169(6):985-99.
- He Xi , Mikhail S, Keiko T, Xin Z. (2004) LDL Receptor-Related Proteins 5 and 6 in Wnt/beta-catenin Signaling: Arrows Point the Way. Development. 131(8):1663-77.
- Bourhis E, Tam C, Franke Y, Bazan JF, Ernst J, et al. (2010) Reconstitution of a frizzled8.Wnt3a.LRP6 signaling complex reveals multiple Wnt and Dkk1 binding sites on LRP6. J Biol Chem. 285(12):9172-9.
- MacDonald BT, He X. (2012) Frizzled and LRP5/6 receptors for Wnt/β-catenin signaling. Cold Spring Harb Perspect Biol. 4(12).
- Larabell CA, Torres M, Rowning BA, Yost C, Miller JR, et al. (1997) Establishment of the dorso-ventral axis in Xenopus embryos is presaged by early asymmetries in beta-catenin that are modulated by the Wnt signaling pathway. Cell Biol. 136(5):1123-36.
- Yost C, Torres M, Miller JR, Huang E, Kimelman D, et al. (1996) The axis-inducing activity, stability, and subcellular distribution of beta-catenin is regulated in Xenopus embryos by glycogen synthase kinase 3. Genes Dev. 10(12):1443-54.
- Zeng L, Fagotto F, Zhang T, Hsu W, Vasicek TJ, et al. (1997) The mouse Fused locus encodes Axin, an inhibitor of the Wnt signaling pathway that regulates embryonic axis formation. Cell. 90(1):181-92.
- Chia IV, Costantini F. (2005) Mouse axin and axin2/conductin proteins are functionally equivalent in vivo. Mol Cell Biol. 25(11):4371-6.
- Spink KE, Polakis P, Weis WI. (2000) Structural basis of the Axin-adenomatous polyposis coli interaction. EMBO J. 19(10):2270-9.
- Behrens J, Jerchow BA, Würtele M, Grimm J, Asbrand C, et al. (1998) Functional Interaction of an Axin Homolog, Conductin, With Beta-Catenin, APC, and GSK3beta. Science. 280(5363):596-9.
- Hart MJ, de los Santos R, Albert IN, Rubinfeld B, Polakis P. (1998) Downregulation of beta-catenin by human Axin and its association with the APC tumor suppressor, beta-catenin and GSK3 beta. Curr Biol. 8(10):573-81.
- Kishida S, Yamamoto H, Ikeda S, Kishida M, Sakamoto I, et al. (1998) Axin, a Negative Regulator of the Wnt Signaling Pathway, Directly Interacts With Adenomatous Polyposis Coli and Regulates the Stabilization of Beta-Catenin. J Biol Chem. 1998 May 1; 273(18):10823-6.
- Hsu SC, Galceran J, Grosschedl R. (1998) Modulation of transcriptional regulation by LEF-1 in response to Wnt-1 signaling and association with beta-catenin. Mol Cell Biol. 18(8):4807-18.
- Willert K, Jeffrey DB, Esther D, Andrew WD, Irving LW, et al. (2003) Wnt proteins are lipid-modified and can act as stem cell growth factors. Nature. 423:448-52.
- Jiang J, Struhl G. (1998) Regulation of the Hedgehog and Wingless signalling pathways by the F-box/WD40-repeat protein Slimb. Nature. 391(6666):493-6.
- Marikawa Y, Elinson RP. (1998) beta-TrCP is a negative regulator of Wnt/beta-catenin signaling pathway and dorsal axis formation in Xenopus embryos. Mech Dev. 77(1):75-80.
- Lagna G, Carnevali F, Marchioni M, Hemmati-Brivanlou A. (1999) Negative regulation of axis formation and Wnt signaling in Xenopus embryos by the F-box/WD40 protein beta TrCP. Mech Dev.; 80(1):101-6.
- Orford K, Crockett C, Jensen JP, Weissman AM, Byers SW. (1997) Serine phosphorylation-regulated ubiquitination and degradation of beta-catenin. J Biol Chem. 272(40):24735-8.
- García de Herreros A, Duñach M. (2019) Intracellular Signals Activated by Canonical Wnt Ligands Independent of GSK3 Inhibition and β-Catenin Stabilization. Cells. 8(10).
- Van de Wetering M, Oosterwegel M, Dooijes D, Clevers H. (1991) Identification and cloning of TCF-1, a T lymphocyte-specific transcription factor containing a sequence-specific HMG box. EMBO J. 10(1):123-32.
- Waterman ML, Fischer W H, Jones KA. (1991) A Thymus-Specific Member of the HMG Protein Family Regulates the Human T Cell Receptor C Alpha Enhancer. Genes Dev. 5(4):656-69.
- Chen CM, Struhl G. (1999) Wingless transduction by the Frizzled and Frizzled2 proteins of Drosophila. Development. 126(23):5441-52.
- Hecht A, Kemler R, Takemaru K I, Moon RT. (2000) The transcriptional coactivator CBP interacts with beta-catenin to activate gene expression. J Cell Biol. 149(2):249-54.
- Monaghan AP, Kioschis P, Wu W, Zuniga A, Bock D, et al. (1999) Dickkopf Genes Are Co-Ordinately Expressed in Mesodermal Lineages. Mech Dev. 87(1-2):45-56.
- Delcommenne M, Tan C, Gray V, Rue L, Woodgett J, Dedhar S. Phosphoinositide-3-OH Kinase-Dependent Regulation of Glycogen Synthase Kinase 3 and Protein Kinase B/AKT by the Integrin-Linked Kinase. Proc Natl Acad Sci U S A. 1998 Sep 15; 95(19):11211-6.
- Zhang Z, Hartmann H, Do VM, Abramowski D, Sturchler-Pierrat C, et al. (1998) Destabilization of beta-catenin by mutations in presenilin-1 potentiates neuronal apoptosis. Nature. 395(6703):698-702.
- Ke J, Harikumar KG, Erice C, Chen C, Gu X, et al. (2013) Structure and function of Norrin in assembly and activation of a Frizzled 4-Lrp5/6 complex. Genes Dev. 27(21):2305-19.
- Cadigan KM, Fish MP, Rulifson EJ, Nusse R. (1998) Wingless repression of Drosophila frizzled 2 expression shapes the Wingless morphogen gradient in the wing. Cell. 93(5):767-77.
- Umbhauer M, Djiane A, Goisset C, Penzo-Méndez A, Riou JF, et al. (2000) The C-terminal cytoplasmic Lys-thr-X-X-X-Trp motif in frizzled receptors mediates Wnt/beta-catenin signalling. EMBO J. 19(18):4944-54.
- Barker N, Hurlstone A, Musisi H, Miles A, Bienz M, et al. (2000) The chromatin remodelling factor Brg-1 interacts with beta-catenin to promote target gene activation. EMBO J. 2001 Sep 3; 20(17):4935-43.
- Kramps T, Peter O, Brunner E, Nellen D, Froesch B, et al. (2002) Wnt/wingless signaling requires BCL9/legless-mediated recruitment of pygopus to the nuclear beta-catenin-TCF complex. Cell. 109(1):47-60.
- Tago K, Nakamura T, Nishita M, Hyodo J, Nagai S, Murata Y, et al. (2000) Inhibition of Wnt Signaling by ICAT, a Novel Beta-Catenin-Interacting Protein. Genes Dev. 14(14):1741-9.
- Daniels DL, William IW. (2002) ICAT Inhibits Beta-Catenin Binding to Tcf/Lef-family Transcription Factors and the General Coactivator p300 Using Independent Structural Modules. Mol Cell. 10(3):573-84.
- Ishitani T, Ninomiya-Tsuji J, Nagai S, Nishita M, Meneghini M, et al. (1999) The TAK1-NLK-MAPK-related pathway antagonizes signalling between beta-catenin and transcription factor TCF. Nature. 399(6738):798-802.
- Ishitani T, Kishida S, Hyodo-Miura J, Ueno N, Yasuda J, et al. (2003) The TAK1-NLK mitogen-activated protein kinase cascade functions in the Wnt-5a/Ca(2+) pathway to antagonize Wnt/beta-catenin signaling. Mol Cell Biol. 23(1):131-9.
- Glinka A, Dolde C, Kirsch N, Huang YL, Kazanskaya O, et al. (2011) LGR4 and LGR5 are R-spondin receptors mediating Wnt/β-catenin and Wnt/PCP signalling. EMBO Rep. 12(10):1055-61.
- Ramírez-Weber FA, Kornberg TB. (1999) Cytonemes: cellular processes that project to the principal signaling center in Drosophila imaginal discs. Cell. 97(5):599-607.
- Greco V, Hannus M, Eaton S. (2001) Argosomes: A Potential Vehicle for the Spread of Morphogens Through Epithelia. Cell. 106(5):633-45.
- Yamazaki Y, Palmer L, Alexandre C, Kakugawa S, Beckett K, et al. (2016) Godzilla-dependent transcytosis promotes Wingless signalling in Drosophila wing imaginal discs. Nat Cell Biol. 18(4):451-7.
- Famili F, Naber BA, Vloemans S, de Haas EF, Tiemessen MM, Staal FJ. (2015) Discrete roles of canonical and noncanonical Wnt signaling in hematopoiesis and lymphopoiesis. Cell Death Dis. 6:e1981.
- Luis TC, Staal FJ. (2009) WNT proteins: environmental factors regulating HSC fate in the niche. Ann N Y Acad Sci. 1176:70-6.
- Ichii M, Frank MB, Iozzo RV, Kincade PW. (2012) The canonical Wnt pathway shapes niches supportive of hematopoietic stem/progenitor cells. Blood. 119(7):1683-92.
- Richter J, Stanley EG, Ng ES, Elefanty AG, Traver D, et al. (2018) WNT9A Is a Conserved Regulator of Hematopoietic Stem and Progenitor Cell Development. Genes (Basel). 9(2). pii: E66.
- Roelink H, Wang J, Black DM, Solomon E, Nusse R. (1993)Molecular cloning and chromosomal localization to 17q21 of the human wnt3 gene. Genomics 17:790-92.
- Duinhouwer LE, Tüysüz N, Rombouts EW, Ter Borg MN, Mastrobattista E, et al. (2015) Wnt3a protein reduces growth factor-driven expansion of human hematopoietic stem and progenitor cells in serum-free cultures. PLoS One. 10(3):e0119086.
- Luis TC, Naber BA, Roozen PP, Brugman MH, de Haas EF, et al. (2011) Canonical wnt signaling regulates hematopoiesis in a dosage-dependent fashion. Cell Stem Cell. 9(4):345-56.
- Famili F, Brugman MH, Taskesen E, Naber BEA, Fodde R, et al. (2016) High Levels of Canonical Wnt Signaling Lead to Loss of Stemness and Increased Differentiation in Hematopoietic Stem Cells. Stem Cell Reports. 6(5):652-59.
- Famili F, Perez LG, Naber BA, Noordermeer JN, Fradkin LG, et al. (2016) The non-canonical Wnt receptor Ryk regulates hematopoietic stem cell repopulation in part by controlling proliferation and apoptosis. Cell Death Dis. 7(11):e2479.
- Osugui L, de Roo JJ, de Oliveira VC, Sodré ACP, Staal FJT, et al. (2018) B-1 cells and B 1 cell precursors prompt different responses to Wnt signaling. PLoS One. 13(6):e0199332.
- Staal FJ, Luis TC, Tiemessen MM. (2008) WNT signalling in the immune system: WNT is spreading its wings. Nat Rev Immunol. 8(8):581-93.
- Pongracz JE, Parnell SM, Jones T, Anderson G, Jenkinson EJ. (2006) Overexpression of ICAT highlights a role for catenin-mediated canonical Wnt signalling in early T cell development. Eur J Immunol. 36(9):2376-83.
- Weerkamp F, Baert MR, Brugman MH, Dik WA, de Haas EF, et al. (2006) Human thymus contains multipotent progenitors with T/B lymphoid, myeloid, and erythroid lineage potential. Blood. 107(8):3131-7.
- Gattinoni L, Xiao-Song Z, Douglas CP, Yun J, Christian SH, et al. (2009) Wnt signaling arrests effector T cell differentiation and generates CD8+ memory stem cells. Nat Med. 15(7): 808-13.
- Luo L, Li Z, Luo G, Zhao Y, Yang J, et al. (2016) Role of Wnt3a expressed by dendritic cells in the activation of canonical Wnt signaling and generation of memory T cells during primary immune responses. Cell Immunol. 310:99-107.
- Van Loosdregt J, Fleskens V, Tiemessen MM, Mokry M, van Boxtel R, et al. (2013) Canonical Wnt signaling negatively modulates regulatory T cell function. Immunity. 39(2):298-310.
- Daniel S, Santhakumar M. Wnt Signaling in Dendritic Cells: Its Role in Regulation of Immunity and Tolerance. Discov Med. 19(105):303-10.
- Suryawanshi A, Raghu K T, Daniel S, Santhakumar M. Modulation of Inflammatory Responses by Wnt/β-Catenin Signaling in Dendritic Cells: A Novel Immunotherapy Target for Autoimmunity and Cancer. Front Immunol. 2016; 7: 460.
- Valencia J, Hernández-López C, Martínez V G, Hidalgo L, Zapata AG, et al. (2011) Wnt5a skews dendritic cell differentiation to an unconventional phenotype with tolerogenic features. J Immunol. 187(8):4129-39.
- Oderup C, LaJevic M, Butcher EC. (2013) Canonical and Noncanonical Wnt Proteins Program Dendritic Cell Responses for Tolerance. J Immunol. (12):6126-34.
- Staal FJ, Luis TC, Tiemessen MM. (2008) WNT signalling in the immune system: WNT is spreading its wings. Nat Rev Immunol. 8(8):581-93.
- Staal FJ, Meeldijk J, Moerer P, Jay P, Van de Weerdt BC, et al. (2001) Wnt signaling is required for thymocyte development and activates Tcf-1 mediated transcription. Eur. J. Immunol. 31:285-93.
- Kared H, Tan SW, Lau MC, Chevrier M, Tan C, et al. (2020) Immunological history governs human stem cell memory CD4 heterogeneity via the Wnt signaling pathway. Nat Commun. 11(1):821.
- Zhanfeng L, Lianjun Z, Huiting S, Rong L, Ning N, et al. (2018) MTOR signaling is essential for the development of thymic epithelial cells and the induction of central immune tolerance. Autophagy. 14(3): 505-17.
- Osada M, Jardine L, Misir R, Andl T, Millar SE,, Millar SE, et al. (2010) DKK1 Mediated Inhibition of Wnt Signaling in Postnatal Mice Leads to Loss of TEC Progenitors and Thymic Degeneration. PLoS One. 5(2):e9062.
- Kvell K, Varecza Z, Bartis D, Hesse S, Parnell S, et al. (2010) Wnt4 and LAP2alpha as pacemakers of thymic epithelial senescence. PLoS One. 5(5):e10701.
- Varecza Z, Kvell K, Talabér G, Miskei G, Csongei V, et al. (2011) Multiple suppression pathways of canonical Wnt signalling control thymic epithelial senescence. Mech Ageing Dev. 132(5):249-56.
- Martínez SF, Ruiz-Mateos E , Dudakov AJ, Velardi E, Grillari J, et al. (2015) WNT Signaling Suppression in the Senescent Human Thymus. J Gerontol A Biol Sci Med Sci. 70(3):273-81.
- Wiekmeijer AS, Pike-Overzet K, IJspeert H, Brugman MH, Wolvers-Tettero IL, et al. (2016) Identification of checkpoints in human T-cell development using severe combined immunodeficiency stem cells. J Allergy Clin Immunol. 137(2):517-526.e3.
- Kirikoshi H, Sekihara H, Katoh M. (2001) WNT10A and WNT6, clustered in human chromosome 2q35 region with head-to-tail manner, are strongly coexpressed in SW480 cells. Biochem. Biophys. Res. Commun. 283: 798-805.
- Hashimi ST, Fulcher JA, Chang MH, Gov L, Wang S, et al. (2009) MicroRNA profiling identifies miR-34a and miR-21 and their target genes JAG1 and WNT1 in the coordinate regulation of dendritic cell differentiation. Blood 114:404-14.
- Lobov IB, Sujata R, Thomas JC, Jefferson EV, Masataka I, et al. (2005) WNT7b mediates macrophage induced programmed cell death in patterning of the vasculature. Nature. 437:417-21.
- Huguet L, McMahon JA, McMahon AP, Bicknell R, Harris AL. (1994) Differential expression of human Wnt genes 2, 3, 4, and 7B in human breast cell lines and normal and disease states of human breast tissue. Cancer Res. 54:2615-621.
- Saitoh T, Hirai M, Katoh M. (2001)Molecular cloning and characterization of Wnt3a and WNT14 clustered in human chromosome 1q42 region. Biochem. Biophys. Res Commun. 284:1168-1175.
- Lako M, Lindsay S, Bullen P, Wilson DI, Robson SC, et al. (1998) A novel mammalian wnt gene, WNT8B, shows brain-restricted expression in early development, with sharply delimited expression boundaries in the developing forebrain. Hum Mol Genet. 7(5):813-22.
- Bui TD, Rankin J, Smith K, Huguet EL, Ruben S, et al. (1997) A novel human Wnt gene, Wnt10b, maps to 12q13 and is expressed in human breast carcinomas. Oncogene. 14:1249-1253.
- Gavin BJ, McMahon JA, McMahon AP. (1990) Expression of multiple novel Wnt-1/int-1-related genes during fetal and adult mouse development. Genes Dev. 4:2319-2332.
- Liang H, Andrew HC, Zhiqing Z, Jennifer Z, Roland J, et al. (2007) Noncanonical Wnt signaling promotes apoptosis in thymocyte development. J Exp Med. 204:3077-3084.
- Liang H, Qin C, Andrew HC, Stephen JA, German P, et al. (2003) Wnt5a inhibits B cell proliferation and functions as a tumor suppressor in hematopoietic tissue. Cancer Cell. 4:349-360.
- Ikegawa S, Kumano Y, Okui K, Fujiwara T, Takahashi E, et al. (1996) Isolation, characterization and chromosomal assignment of the human wnt7a gene. Cytogenet Cell Genet. 74:149-152.
- Bergstein I, Eisenberg LM, Bhalerao J, Jenkins NA, Copeland NG, et al. (1997)Isolation of two novel WNT genes, WNT14 and WNT15, one of which (WNT15) is closely linked to WNT3 on human chromosome 17q21. Genomics. 46:450-458.