The Effects of Stem Cells on Recovery after Stroke
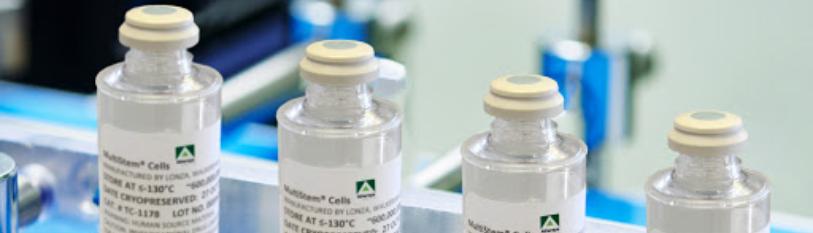
Linsey Yarbrough and Vincent S Gallicchio*
Department of Biological Sciences, College of Science, Clemson University, Clemson, USA
*Corresponding author: Vincent S Gallicchio, Department of Biological Sciences, College of Science, Clemson University, Clemson, USA
Citation: Yarbrough L, Gallicchio VS. (2020) The Effects of Stem Cells on Recovery after Stroke. J Stem Cell Res. 1(1):1-16.
Received: July 13, 2020, | Published: July 30, 2020
Copyright© 2020 genesis pub by Yarbrough L. CC BY-NC-ND 4.0 DEED. This is an open-access article distributed under the terms of the Creative Commons Attribution-Non Commercial-No Derivatives 4.0 International License., This allows others distribute, remix, tweak, and build upon the work, even commercially, as long as they credit the authors for the original creation.
DOI: https://doi.org/10.52793/JSCR.2020.1(1)-06
Abstract
Stroke is a prevalent cause of death and disability worldwide with a limited time window for treatment. The current treatment methods must be administered within a few hours after the onset of stroke. Embryonic Stem Cells (ESCs), Induced Pluripotent Stem Cells (iPSCs), Neural Stem Cells (NSCs), Mesenchymal Stem Cells (MSCs), Dental Pulp Stem Cells (DPSCs), and Autologous CD34+ Stem Cells have all shown promise as potential therapies after stroke due to regenerative, anti-inflammatory, multi-differentiation, and neuroprotective properties when tested after transplantation in animals. Athough the safety of NSCs, MSCs, and Autologous CD34+ Stem Cells has been shown through human clinical trials; additional clinical studies must be conducted in order to determine the efficacy of stem cell infusion after stroke.
Keywords
Stem cells; Stroke; Rehabilitation; Recovery; Ischemia
Introduction
Stroke is the second most prevalent cause of death worldwide under heart disease [1,4,15,16,39]. It is also the primary cause of disability in the United States with more than 700,000 Americans are affected by stroke each year [1]. There is still uncertainty about the progression and recovery of stroke, but risk factors include family history of stroke, age, sex, hypertension, hypercholesterolemia, and smoking [2]. Initial symptoms of stroke include paralysis, impaired speech, and vision loss [2]. Stroke is a cerebrovascular disease which occurs due to a disruption in blood supply to the brain causing the loss of neurons, astroglia, and oligodendria as well as disruption of blood vessels [3,12]. Neural cell death is accompanied by an increase in apoptosis, a depletion of calcium and energy, along with inducing an inflammatory response [3].
There are two types of stroke: intracranial hemorrhages, which make up roughly 13%, and ischemic stroke which make up the other 87% [4,12]. Ischemic stroke includes lacunar, territorial and distal field infarctions. Lacunar infarction typically involves arteries of the basal ganglia and brainstem, while territorial infarction involves occlusions of the carotid or vertebrobasilar system. Distal filed infarctions stretch between two or three adjacent arterial territories [4]. Following ischemic stroke, inflammatory response of the brain has been shown to disrupt the blood-brain barrier (BBB). Blood derived substances are then able to penetrate into the brain which leads to edema and disruption of neuronal function [5,6]. During the first few hours after an ischemic stroke, cytokines and chemokines are released, some of which are pro-inflammatory which increases the infarct size and amplifies injury [5-7]. These pro-inflammatory mediators can have positive effects on neurogenesis, but prolonged inflammation can lead to a decrease in regenerative processes and viable neurons [7].
Current treatment for stroke includes thrombolysis, thrombectomy, and rehabilitation therapy [3]. Thrombolysis became the recommended treatment for patients based on a trial conducted in 1995, but only if patients were treated within three hours of the ischemic stroke [4]. Thrombolysis is the use of thrombolytic drugs to destroy the blood clot and restore blood flow before further damage can be done [8]. One of the thrombolytic drugs that has been proven to be effective is alteplase, which is a recombinant tissue plasminogen activator (r-tPA) that converts plasminogen to plasmin [9,10]. Thrombolytics are still given to patients within a three-hour time window; however, they have been seen to cause significant brain bleeding [8]. Thrombectomy has been shown to reduce the severity of disability after stroke, but a study done in 2014 showed that it must be done within an 8-hour time window [10]. Recently, thrombectomy has revolutionized acute stroke treatment as it can be applied in patients for up to 24 hours. However, the number of patients that qualify for the treatment remains low [11]. Thrombolysis and thrombectomy have both been shown to reduce disability and prevent further damage, but both must be done within a small-time window. Additionally, once ischemic stroke damage has been done to the brain, there is not much that can be done to repair it [9]. Therefore, stem cells have been tested as a potential regenerative treatment after stroke [3]. Embryonic stem cells (ESCs), Induced pluripotent stem cells (iPSCs), Neural stem cells (NSCs), Mesenchymal stem cells (MSCs), Dental pulp stem cells (DPSCs), and Autologous CD34+ stem cells have been tested for their effects on ischemic stroke damage [3].
Embryonic stem cells (ESCs) are derived from the inner cell mass of a human embryo, and are pluripotent with the ability to differentiate into virtually any type of cell. ESCs have the ability to differentiate into neural lineage under certain conditions. ESCs possess limitations including ethical concerns and potential tumor transformation [3]. Since human embryonic stem cells (hESCs) offer the potential to differentiate into almost any cell type they could serve as an unlimited source of neural cells, which could result in a source for repair in stroke [12]. ESCs have been found to generate a heterogeneous population of cells when derived by direct enrichment. This heterogeneous population, however, could contain undifferentiated stem cells that could cause the formation of tumors [12].
Induced pluripotent stem cells (iPSCs) have been viewed as a potential way of overcoming the ethical concerns that arise with embryonic stem cells [13]. Induced pluripotent stem cells are derived from differentiated cells and are pluripotent due to transfection with transcription factors [14]. These cells resemble ESCs through their morphology and growth properties, but avoid ethical problems that arise with ESCs [3]. iPSCs can also be generated from the patient’s own cells, which avoids the potential of the immune system to reject the transplanted cells [15]. Human iPSCs can be easily tracked in animal models with the use of magnetic resonance imaging (MRI) [14]. However, iPSCs also run the risk of possibly leading to potential tumor formation [16].
Another type of stem cells being investigated for potential stroke treatment are Neural stem cells (NSCs), which can be delivered either by endogenous or exogenous methods. The endogenous approach uses stem cells that already exist within the individual and attempts to stimulate their growth, mobilization, and stability [16]. Human NSCs are found in the subventricular zone (SVZ), the olfactory bulb, and the dentate gyrus of the hippocampus. Endogenous NSCs produce neurotrophic factors, which allow them to regulate the environment and inflammatory responses happening within it [3]. The exogenous approach uses the transplantation of NSCs that have been obtained from a different source. These NSCs have the ability to migrate into the damaged ischemic tissue, and have the potential to produce an unlimited source of neural cells [16]. Exogenous NSCs have been shown to differentiate into neurons, astrocytes, and oligodendrocytes in vitro (3). In previous studies, a minimal amount of transplanted NSCs have been seen to survive and integrate successfully into the ischemic brain (Figure 1).
Figure 1: Represents the mechanism by which exogenous NSCs are transplanted and can be differentiated into NSCs, migrate into the cerebrospinal fluid, or serve as a modulator for immune response [16].
Mesenchymal stem cells (MSCs) have also been investigated because they have the ability to be isolated from almost all tissues in mammals. MSCs have also been shown to differentiate into neural cells and to migrate toward the ischemic brain [3]. Since MSCs are non-tumorigenic and show no ethical issues and concerns with tissue rejection, they show promise for clinical therapy after stroke [3,27]. Their therapeutic effects are mediated through paracrine activity by their trophic factors [15]. MSCs have also shown immunomodulatory properties in which they inhibit lymphocytes and microglia in order to modulate immune response of cytokine secretion [7].
Dental pulp stem cells (DPSCs) are another cell population of interest as they are easily attainable. DPSCs originate from the neural crest and exhibit neuro-ectodermal features and contain potential to have multi-directional differentiation. DPSCs can be isolated during routine dental procedures without bringing up ethical concerns (11). They can also be obtained from teeth that have been removed and discarded as waste (17). DPSCs can also retain their properties after being cryopreserved for up to a month, which would impact therapeutic stem cell properties of other adult cells [11]. DPSCs have also been found to express NSC associated markers such as Nestin and Sox2 as well as markers associated with mature cell types including several neuronal markers. This shows that DPSCs have the ability to differentiate into neuron-like cells under certain conditions [11].
The last group of stem cells are CD34+ stem cells, which are a part of bone marrow mononuclear cells (BMMNCs) and serve as the major endogenous source of endothelial and hematopoietic cell precursors. Mobilization of CD34+ from bone marrow to the periphery increases after a stroke [18]. CD34+ cells have been found to promote angiogenesis through the secretion of angiogenic factors such as VEGF, bFGF, and HGF [19].
Previous trials that have tested the effects of stem cells on ischemic stroke damage induced in animals show promise for use in humans. Several clinical trials have been conducted to test the safety of stem cell use in humans. This review discusses stem cells as a potential repair mechanism for stroke and previous trials in animals and humans that have been conducted.
Discussion
Animal stem cell treatment
Embryonic Stem Cells
Embryonic stem cells (ESCs) have been shown to differentiate into neural cells; however, there has been concern that these cells may contribute to the formation of tumors. A study was conducted to look at an alternative method of deriving neural cells in order to avoid tumor formation, thus demonstrating the further potential of functional recovery after stroke [12]. Daadi, Maag, and Steinberg tested an alternative method for isolating human neural stem cells (hNSCs) from hESCs. The hESCs were isolated and grown on a mouse feeder layer in a media that contained bFGF. The initial population showed a stable increase and hNSCs had neural precursor cell markers [12]. When mitogenic factors were removed and the hNSCs were plated with poly-L-ornithine substrate, they spontaneously differentiated into neurons, astrocytes, and oligodendrocytes [12]. These SD56 hNSCs were transplanted subcutaneously into the forebrain of rats, and were found to be non-tumorigenic [12]. The hNSCs were also transplanted into a rat with a middle cerebral artery occlusion (MCAO), mimicking stroke injury. The grafted cells survived and migrated into the ischemic tissue [12]. The transplantation of SD56 hNSCs also improved the use of a forelimb that was impaired due to the stroke [12]. Scientists concluded that this hNSC line could serve as a source of neural cells for recovery after stroke without tumor formation [12] (Figure 2).
Figure 2: This graph compares the use of the injured forelimb in rats before and after transplantation of hNSCs and injection of vehicle (control). There was a significant increase in the use of the impaired contralateral forelimb at 4 and 8 weeks after hNSC transplantion but not vehicle injection (12).
In another study, Oyamada et al. plated human embryonic stem cells which differentiated into embryonic cells and mural cells [19]. The mural cells (hES-MCs) were used for transplantation into a MCAO in mice. Scientists demonstrated that the hES-MCs expressed several angiogenic factors, and improved cerebral blood flow [19]. It was concluded that MCs derived from human ESCs show potential for a regenerative therapy after stroke in humans [19].
Induced Pluripotent Stem Cells
Induced pluripotent stem cells (iPSCs) have also been tested in animals to look at the potential use of iPSCs as a recovery mechanism for stroke. Jiang, et al. tested the effects of iPSCs in rats to see the possibility of iPSCs as a clinical treatment after stroke [13]. They generated iPSCs by taking samples of human fibroblasts and introducing transcription factors Oct4, Sox2, Nanog, and Lin-28. As done in the ESC studies, a middle cerebral artery occlusion (MCAO) was induced on thirty rats. The iPSCs were able to differentiate in vitro and in vivo into the three germ layers [13]. The iPSCs were transplanted into the ischemic area, and the iPSCs were able to survive and migrate into the affected area. Furthermore, the iPSCs were able to differentiate into neural cells, which was shown by the cells that were labeled with the GFAP antibody, and cells positive for Nestin and Vimentin [13]. The transplantation of iPSCs also showed potential to improve sensorimotor function, and researchers overall concluded the iPSCs show promise for clinical treatment [13].
Chau, et al. focused on the potential of iPSCs as a recovery therapy for neonatal/perinatal stroke [20]. The iPSCs expressed Sox2 and Oct3/4 using a retinoic acid protocol to differentiate iPSCs into neural proginitor cells (iPSC-NPCs). The iPSC-NPCs expressed several trophic factors in vitro and the expression of the trophic factors SDF-1a and VEGF increased after transplantation into the ischemic brain (Figure 3) [20].
Figure 3: Trophic factor expression was increased after transplantation of iPSCs into the ischemic brain. SDF-1a and VEGF were both expressed more in the stroke + iPSC groups than the sham (control) and stroke only groups.
Both of these growth factors play a role in migration of neural proginitor cells as well as recruitment of other stem cells. VEGF also increasead angiogenesis so researchers concluded that iPSC-NPC transplantation can lead to regeneration and functional recovery [20].
In two studies induced pluripotent stem cell derived neural progenitor/stem cells (iNPCs/iNSCs) were transplanted into pigs to examine the effects of iPSC transplantation in larger animals [21,22]. These studies used pigs to test the potential therapeutic use of iPSCs in an animal with a brain size much more similar to that of a human [21,22]. Baker, et al. transplanted iNSCs into the ischemic region of pigs after the iNSCs were assessed in vitro [21]. Lau, et al. conducted an MCAO on eight 6-month old pigs and injected them with iNPC five days after stroke [22]. Both studies found that iNSCs/iNPCs expressed neural proginetor markers SOX1 and Nestin and differentiated into neural cells in vitro [21,22]. Baker, et al. concluded that iNSC transplantation into pigs promoted neurogenesis, reduced microglia to slow inflammation, and improved white matter integrity and cerebral perfusion [21]. Lau, et al. concluded that pigs treated with iNPCs had a faster functional recovery compared to the control pigs [22]. Both studies concluded that iNSC/iNPCs show potential for a therapeutic mechanism after stroke in humans [21,22].
Following these studies, Jeong, et al. used iPSCs derived from episomal plasmids to differentiate neural precursor cells (ep-iPSC-NPCs). The ep-iPSC-NPCs were injected into rats 7 days after MCAO was conducted and neurological deficits were confirmed ]15\. Rats that underwent transplantation of ep-iPSC-NPCs showed greater functional recovery compared to the rats in the fibroblast or vehicle groups. The ep-iPSC-NPCs also differentiated into neural cells, enhanced neurogenesis, and reduced inflammation. Researchers concluded that ep-iPSC-NPCs show promise in treatment for stroke patients [15].
Neural Stem Cells
As previously mentioned, Neural stem cells (NSCs) are another readily available source of stem cells that have been tested for potential therapeutic application in stroke, and can be extracted endogenously or exogenously [2]. One study conducted looked at transplantantion of exogenous NSCs. Photothrombotic ischemia was conducted in mice, and NSCs were transplanted at a depth of 3 mm. The results showed that transplantation of NSCs improved brain function and repaired damaged brain tissue [2]. The infarct size of the mice brains was reduced from 120 x 109/L to 60 x 109/L (Figure 4).
Figure 4: The Nissl method was used to stain the brain slices of mice with and without NSC transplantation in order to visualize the difference in neuronal degradation and necrosis. This figure shows the quantified difference in neuronal degradation and necrosis between the control and NSC transplantation groups [2]. Due to the improved brain function and reduction in brain tissue damage, it was concluded that exogenous NSC transplantation shows promise for a therapy after stroke [2].
Another study looked at the endogenous approach to see if human NSCs (hNSCs) would promote endogenous neurogenesis and angiogenesis if transplanted directly into the subventricular zone [23]. The transplanted hNSCs were found to survive, migrate toward the ischemic region, differentiate into neural cells, and both improve functional recovery, and decrease infarct size. The hNSCs did promote neurogenesis and angiogenesis within the SVZ, which was demonstrated for the first time through intraventricular transplantation in rats. Ryu, et al. concluded that the enhanced neurogenesis and angiogenesis show potential for NSCs as a treatment after stroke, but that further studies must be conducted to look at the mechanisms and functional outcome [23].
In April of 2018, a study was conducted to look at human neural stem cell extracellular vesicles (NSC EVs) transplanted in a pig model after recommendations were given by the Stroke Therapy Academic Industry Roundtable and the Stem Cell Emerging Paradigm in Stroke consortium meeting [24]. These recommendations were encouraging testing for a regenerative therapy, and testing done in an animal with a more similar pathology to humans to look at routes of administration and behavior effects. The NSC EVs were transplanted in pigs because both human and porcine brains are gyrencephalic and are made up of more than 60% white matter whereas rodents are made up of less than 10% white matter and are lissencephalic. There were 9 pigs in the control group and 7 in the treated group, and the efficacy of NSC EVs in a porcine MCAO model was evaluated using magnetic resonance imaging (MRI). NSC EV transplantation led to reduced infarct volume, decreased swelling, promoted white matter integrity, and improved function. This led researchers to conclude that NSC EVs show potential to be translated into clinical therapy for human stroke [24].
NSCs have also shown an anti-inflammatory capacity in addition to enhancing neurogenesis. A study compared the immune response in mice with and without transplantation of NSCs [25]. The group with NSCs had a decrease in the number of MAP1LC3-positive and Beclin-1-positive immunoreactive puncta. NSCs also decreased the number of GFAP and IBA1-expressing cells indicating that NSCs have immunomodulatory properties. Lastly, NSCs decreased L-selecting, leptin, MCP-1, and TNFa production indicating they were able to modulate inflammation. Researchers concluded that further investigation should be done to look at the promise that NSCs show for a therapeutic strategy to reduce injury from ischemic stroke [25].
In April 2020, a study revealed that there is a hypersynchronicity of the functional neural networks after mild cortical strokes [26]. Furthermore, the effect of stem cells on the functional networks was also observed. The distal MCA occlusion model was conducted on the mice and hNSCs were implanted next to the lesion. Over a 12-week period, stabilization of the functional networks was found in mice with hNSCs. Researchers concluded that this stabilization induced by hNSCs leads to expectation for clinical application of stem cell therapy after stroke [26].
Mesenchymal Stem Cells
The nontumorigenic and easily accessible properties of mesenchymal stem cells (MSCs) has allowed them to be considered as another potential treatment for stroke. One study looked at the effects of mesenchymal stem cell-derived extracellular vesicles (MSC-EVs) compared to MSCs that were delivered to mice with focal cerebral ischemia [27]. Motor coordination, histology, immune response, and cerebral angiogenesis and neurogenesis were analyzed for 28 days after ischemia. The study showed that MSC-EVs and MSCs improve neurological impairment similarly after ischemia. Since EVs have been reported to be well tolerated in humans, researchers predicted that MSC-EVs could be translated into human patients [27].
Another study isolated human muse cells from human bone marrow MSCs (BM-MSCs) and transplanted either MACS-sorted muse-rich cells, serum-free MSCs, or vehicle PBS into mice with lacunar infarcts. MSCs have been found to provide neuroprotection, anti-inflammatory effects, and induce behavorial recovery; however, muse cells showed a greater benefit especially when transplanted in the late phase of stroke [28]. It was found that muse cells differentiated into neural cells, facilitated pyramidal reconstruction, improved function, and proved to be safe. Researchers discussed the potential of muse cells and MSCs to have a synergistic effect on enhancing functional outcomes of stroke therapy. The study concluded muse cells also show potential for therapy after lacunar stroke [28].
As mentioned, MSCs also have an anti-inflammatory effect to control inflammation after stroke. One study looked at the effects of MSCs on hypoxia-ischemic brain injury, focusing on the immunomodulatory properties [7]. Human placenta amniotic membrane-derived mesenchymal stem cells (AMSCs) were tested on rats with hypoxic-ischemic injuries. AMSCs in a hypoxic environment showed an increase in expression of TGF-b, IL-10, IL-4, and CD200, which encode for anti-inflammatory factors. CD200 is particularly important in modulating immune response by suppressing myeloid cell function. When transplanted into the ischemic boundary zone, AMSCs decreased inflammation in the rat model by suppressing pro-inflammatory cytokine production. When the mRNA expression of pro-inflammatory and anti-inflammatory factors were analyzed, the AMSCs exhibited increased levels of anti-inflammatory factors and a reduced change in pro-inflammatory factors compared to the control group (Figure 5).
Researchers concluded that the supression of pro-inflammatory cytokines by AMSCs in brain ischemia prove MSCs originating in the placenta to be a potential therapy for stroke (7). Another study was conducted to determine if IL-1a-primed MSC-derived secretomes (aCM) are effective on functional recovery after ischemia. The aCM were found to decrease lesion volume by about 30% and improve behavior recovery in mice with ischemia separately from neuroprotective effects. Researchers concluded that aCM has potential to provide a method of acellular therapy as a treatment for ischemic stroke (29).
Figure 5: The mRNA expression of anti-inflammatory and pro-inflammatory factors were looked at after control and AMSC transplantation at 1 day and 1week. a-d show that CD200, IDO, IL-10, and TGF-b anti-inflammatory facters were all expressed higher in rats with AMSC transplantation than after the control treatment. e-i show that IFN-g, TFN-a, IL-1, MCP-1, and GRO-a pro-inflammatory factors were lower in rats with AMSC transplantation than for the control treatment [7].
Dental Pulp Stem Cells
Dental pulp stem ells (DPSCs) are another attractive method for therapy after ischemic brain injury. Song, et al. compared the effectiveness of human DPSCs (hDPSCs) to human bone marrow-derived MSCs (hBM-MSCs) as therapy for cerebral ischemia (30). Researchers investigated intravenous (IV) injection of hDPSCs to look at differentiation, migration, and functional effects after stroke in rats with MCAO as compared to rats transplanted with hBM-MSCs. The DPSCs were obtained from human dental pulp tissues from the third molars or premolars that had been extracted from a patient during a dental procedure. Human MSCs were obtained from isolating them from bone marrow aspirate [30] (Figure 6).
Figure 6: This graph compares the size of the infarct in the control, hDPSC-transplanted, and hBM-MSC-transplanted rats. The severity of neurological functions using the modified neurological severity score (mNSS) and the coronal sections were also compared [30].
Researchers concluded that IV transplantation of hDPSCs show greater decrease in infarct volume as well as comparable functional recovery to transplantation of hBM-MSCs. Therefore, hDPSCs show potential for a new way of using stem cells as a treatment for ischemic stroke [30]. Another group also stated that DPSCs exhibit proliferation three times greater than BM-MSCs in addition to having immunosuppressive properties. They conducted a study to look at the effect of intravascular transplantation of human DPSCs in rats with MCAO with hopes to translate human DPSCs for clinical use. This administration is minimally invasive and the DPSCs were able to cross through the blood brain barrier into the ischemic area proving to be safe and effective. The infarct volumes in rats transfected with DPSCs were reduced by 30% and motor function was also improved. The DPSC-transplant group also showed lower pro-inflammatory cytokine levels and less neuronal degeneration. Researchers concluded that DPSCs do show potential for therapy in the acute phase of ischemic stroke [17[. Zhang, et al. similarly showed that DPSCs can survive, migrate into the ischemic area, and express neuronal markers [31].
Furthermore, researchers looked at the therapeutic potential of DPSCs with brain-derived neurotrophic factor (BDNF) in focal cerebral ischemia [32]. Rats with MCAO were randomly put into either the BDNF, DPSCs, DPSCs+BDNF, or saline (control) group. BDNF is part of the neurotrphin growth factor family and acts to exert trophic effects, protect neurons, and plays a role in growth and differentiation. DPSCs combined with BDNF enhanced neurological recovery through increasing the viability of the transplanted cells and enhancing cell differentiation and neuroprotection when compared to the groups with DPSCs or BDNF alone. Researchers concluded that DPSCs together with BDNF show promise for a clinical therapy for cerebral ischemia [32].
CD34+ Stem Cells
CD34+ stem cells have been examined for their way of accelerating neovascularization. One study administered human CD34+ cells, CD34- cells, or PBS to mice 48 hours after stroke [33]. Mice treated with CD34- or PBS did not show improvements in functional recovery, neurogenesis, or neovascularization. However, CD34+ cells enhanced neovascularization in the ischemic zone. Following neovascularization, endogenous neurogenesis and functional recovery were improved. Scientists concluded CD34+ cells are necessary for neovessel formation, which leads to neuronal regeneration after stroke, which could explain the therapeutic effects that are achieved through transplantation of neuronal cells (33).
Clinical Trials
Since stem cell research is relatively new, only a few clinical trials have been conducted in humans to look at the effects of stem cells after stroke. The efficacy is still unknown, but some studies that have been conducted on humans show the safety and potential of the use of stem cells as a therapy after stroke [34].
The first study to look at hNSCs in humans after stroke was started in 2013 after 11 men were recruited [34]. The men who received treatment were all age 60 or older and had an ischemic stroke within 6-60 months with a minimum infarct of 1 cm in diameter on MRI. CTX0E03 hNSCs were injected into patients and imaging data was collected for 2 years following injection. Researchers found that injection of these stem cells did not lead to any adverse events and were related to improvement in neurological function. It was concluded that injection of CTX0E03 hNSCs is a feasible and safe therapy for stroke, and that a larger phase 2 trial should be conducted [34].
Human MSCs were also infused into humans in a study conducted on patients ranging from ages 41 to 73 years who had a stroke within the last 6 months [35]. There was a reduction in NIHSS score of at least four points in 7 of the 12 pateints, and this improvement in the National Institutes of Health Stroke Scale (NIHSS) score seemed to correlate with a change in lesion volume (Figure 7).
Figure 7: A shows lesion volumes found from MRI pre-infusion of MSCs, 1 day, 2 days, 1 week, and 2 weeks post-infusion of MSCs.
B shows the percent reduction in lesion volume.
C shows the mean volume percent change of the lesion [35].
Researchers concluded that in order to determine the efficiency of human MSCs as a treatment for stroke, more studies need to be conducted to look at overall function and to determine proper protocol. Their results support the safety of human MSCs cultured from autologous human serum; however, more blinded studies with larger sample sizes were recommended by researchers [35].
Another study was conducted by Lee, et al. to test the safety of MSC transplantation in patients [36]. The clinical trial was observer-blinded and consisted of patients with severe middle cerebral artery territory infarcts who were followed for five years. Patients were randomly selected to be in the MSC group or the control group and 52 of the 85 patients were included in the study. Researchers concluded that administering the autologous MSCs through IV application is feasible and safe [36]. Functional recovery was observed more in patients in the MSC group than in the control group. However, scientists mentioned the limitations of the study including the small number of participants and a potential placebo effect since the trial was not double-blinded [36]. In order to further assess the efficacy of MSCs in patients, more trials should be conducted with larger sample sizes.
Clinical trials were also conducted to look at intra-arterial immunoselected CD34+ stem cells administered after acute ischemic stroke [37]. The first was conducted in 2014 and found that intra-arterial infusion of CD34+ selected cells within 7 days of stroke was safe [37]. Another clinical study was conducted to look at the safety and efficacy of autologous stem cells infused intra-arterially after subacute ischemic stroke (38). The study included 20 patients of which 10 were given the intra-arterial CD34+ stem cell infusion 8-15 days after stroke and were analyzed after 1, 3, and 6 months. Researchers concluded that the study showed that the intra-arterial infusion of autologous stem cells is safe. There was also a trend toward improvement in clinical outcome in patients with CD34+ cell treatment compared to the control group; however, researchers stated that the study was too small to make any clinical benefit claims. Larger randomized studies were suggested in order to validate the findings of the study [38].
Conclusion
The current therapies for stroke include intravenous tissue plasminogen activator and endovascular therapy. However, both of these therapies have a limited time window that they can be administered after the onset of stroke (38). Infusion of stem cells shows promise for therapy after stroke. ESCs, iPSCs, NSCs, MSCs, DPSCs, and CD34+ stem cells have all shown efficacy during trials conducted on animals. Although the efficacy of stem cells has not been proven in humans, the safety of NSCs, MSCs, and CD34+ stem cells has been shown through human clinical trials [35-39]. More trials are encouraged using larger sample sizes to look at the efficacy and functional effects of stem cell transplantation on patients after stroke [35-39].
References
- Jiang M, Lv L, Ji H, Yang X, Zhu W, et al. (2011) Induction of pluripotent stem cells transplantation therapy for ischemic stroke. Mol Cell Biochem. 354(1-2):67-75.
- Hou B, Ma J, Guo X, Ju F, Gao J, et al. (2017) Exogenous Neural Stem Cells Transplantation as a Potential Therapy for Photothrombotic Ischemia Stroke in Kunming Mice Model. Mol Neurobiol. 54(2):1254-62.
- Hao L, Zou Z, Tian H, Zhang H, Zhou H, et al. (2014) Stem Cell-Based Therapies for Ischemic Stroke. BioMed Res Intern. 2014:1-17.
- Stapf C, Mohr JP. (2002) Ischemic Stroke Therapy. Annu Rev Med. 53(1):453-75.
- Yang C, Hawkins KE, Dore S, Jalil EC. (2019) Neuroinflammatory mechanisms of blood-brain barrier damage in ischemic stroke. Am J Physiol Cell Physiol. 316(2):C135-C153.
- Jiang X, Andjelkovic AV, Zhu L, Yang T, Bennett MVL, et al. (2018) Blood-brain barrier dysfunction and recovery after ischemic stroke. Prog Neurobiol. 163-164:144-171.
- Kong T, Park JM, Jang JH, Kim Cy, Bae CH, et al. (2018) Immunomodulatory effect of CD200-positive human placenta-derived stem cells in the early phase of stroke. Exp Mol Med. 50(1):e425.
- Wardlaw Joanna M, Murray V, Berge E, J del Zoppo G. (2014) Thrombolysis for Acute Ischaemic Stroke. Cochrane Database Syst Rev. 2014(7):CD000213.
- Fisher M, Jeffrey LS. (2015) Future Directions of Acute Ischaemic Stroke Therapy. Lancet Neurol. 4(7):758-67.
- Jovin Tudor G, Chamoroo A, Cobo E, de Miquel MA, Molina CA, et al. (2015) Thrombectomy within 8 Hours after Symptom Onset in Ischemic Stroke. N Engl J Med. 372:2296-306.
- Lan X, Sun Z, Chu C, Boltze J, Li S. (2019) Dental Pulp Stem Cells: An Attractive Alternative for Cell Therapy in Ischemic Stroke. Front Neurol. 10:824.
- Daadi MM, Maag AL, Steinberg GK. (2008) Adherent self-renewable human embryonic stem cell-derived neural stem cell line: functional engraftment in experimental stroke model. PLoS One. 3(2):e1644.
- Jiang M, Lv L, Ji H, Yang X, Zhu W, et al. (2011) Induction of pluripotent stem cells transplantation therapy for ischemic stroke. Mol Cell Biochem. 354(1-2):67-75.
- Surugiu R, Olaru A. (2019) Recent Advances in Mono- and Combined Stem Cell Therapies of Stroke in Animal Models and Humans. Int J Mol Sci. 20(23): 6029.
- Oh SH, Jeong YW, Choi W, Noh JE, Lee S, et al. (2020) Multimodal Therapeutic Effects of Neural Precursor Cells Derived from Human-Induced Pluripotent Stem Cells through Episomal Plasmid-Based Reprogramming in a Rodent Model of Ischemic Stroke. Stem Cells Int. 2020:4061516.
- Bernstock Joshua D, Jametti LP, Ye D, Gessler FA, Maric D, et al. (2017) Neural stem cell transplantation in ischemic stroke: A role for preconditioning and cellular engineering. J Cereb Blood Flow Metab. 37(7):2314-19.
- Nito C, Sowa K, Nakajima M, Sakamoto Y, Suda S, et al. (2018) Transplantation of Human Dental Pulp Stem Cells Ameliorates Brain Damage Following Acute Cerebral Ischemia. Biomed Pharmacother. 108:1005-1014.
- Banerjee, Soma, et al. Intra‐Arterial Immunoselected CD34+ Stem Cells for Acute Ischemic Stroke
- Oyamada N, Itoh H, Sone M. (2008) Transplantation of vascular cells derived from human embryonic stem cells contributes to vascular regeneration after stroke in mice. J Transl Med. 6:54.
- Chau Monica J, Deveau TC, Song M, Gu S, Chen D, et al. (2014) IPSC Transplantation Increases Regeneration and Functional Recovery After Ischemic Stroke in Neonatal Rats. Stem Cells. 32(12):3075-87.
- Chau MJ, Deveau TC, Song M, Gu X, Chen D, Wei L. (2014) iPSC transplantation increases regeneration and functional recovery after ischemic stroke in neonatal rats. Stem Cells. 32(12):3075-87.
- Baker EW, Platt SR, Lau VW, Grace HE, Holmes SP, et al. (2017) Induced pluripotent stem cell-derived neural stem cell therapy enhances recovery in an ischemic stroke pig model. Sci Rep. 7(1):1-5.
- Lau VW, Platt SR, Grace HE, Baker EW, West FD. (2018) Human iNPC therapy leads to improvement in functional neurologic outcomes in a pig ischemic stroke model. Brain Behav. 8(5):e00972.
- Ryu S, Lee SH, Kim SU, Yoon BW. (2016) Human neural stem cells promote proliferation of endogenous neural stem cells and enhance angiogenesis in ischemic rat brain. Neural Regen Res. 11(2):298.
- Webb RL, Kaiser EE, Jurgielewicz BJ, Spellicy S, Scoville SL, et al. (2018) Human neural stem cell extracellular vesicles improve recovery in a porcine model of ischemic stroke. Stroke. 49(5):1248-56.
- Yu X, Wang X, Zeng S, Tuo X. (2018) Protective effects of primary neural stem cell treatment in ischemic stroke models. Exp Ther Med. 16(3):2219-28.26.
- Minassian A, Green C, Diedenhofen M, Vogel S, Hess S, et al. (2020) Human Neural Stem Cell Induced Functional Network Stabilization After Cortical Stroke: A Longitudinal Resting-State fMRI Study in Mice. FRONT CELL NEUROSCI. 14:86.
- Doeppner TR, Herz J, Görgens A, Schlechter J, Ludwig AK, et al. (2015) Extracellular vesicles improve post‐stroke neuroregeneration and prevent postischemic immunosuppression. Stem Cells Transl Med. 4(10):1131-43.
- Uchida H, Niizuma K, Kushida Y, Wakao S, Tominaga T, et al. (2017) Human Muse cells reconstruct neuronal circuitry in subacute lacunar stroke model. Stroke. 48(2):428-35.
- 29. Cunningham CJ, Wong R, Barrington J, Tamburrano S, Pinteaux E, et al. (2020) Systemic conditioned medium treatment from interleukin-1 primed mesenchymal stem cells promotes recovery after stroke. STEM CELL RES THER. 11(1):32.
- Song M, Lee JH, Bae J, Bu Y, Kim EC. (2017) Human dental pulp stem cells are more effective than human bone marrow-derived mesenchymal stem cells in cerebral ischemic injury. Cell Transplantation. 26(6):1001-16.
- Zhang X, Zhou Y, Li H, Wang R, Yang D, et al. (2018) Transplanted dental pulp stem cells migrate to injured area and express neural markers in a rat model of cerebral ischemia. CELL PHYSIOL BIOCHEM. 45(1):258-66.
- Zhang X, Zhou Y, Li H, Wang R, Yang D, et al. (2018) Intravenous administration of DPSCs and BDNF improves neurological performance in rats with focal cerebral ischemia. Int J Mol Med. 41(6):3185-94.
- Taguchi A, Soma T, Tanaka H, Kanda T, Nishimura H, et al. (2004) Administration of CD34+ cells after stroke enhances neurogenesis via angiogenesisin a mouse model. J CLIN INVEST. 114(3):330-8.
- Kalladka D, Sinden J, Pollock K, Haig C, McLean J, et al. (2016) Human neural stem cells in patients with chronic ischaemic stroke (PISCES): a phase 1, first-in-man study. The Lancet. 388(10046):787-96.
- Honmou O, Houkin K, Matsunaga T, Niitsu Y, Ishiai S, et al. (2011) Intravenous administration of auto serum-expanded autologous mesenchymal stem cells in stroke. Brain. 134(6):1790-807.
- Lee JS, Hong JM, Moon GJ, Lee PH, Ahn YH, et al. (2010) A long-term follow-up study of intravenous autologous http://stroke. ahajournals. org/Downloaded from mesenchymal stem cell transplantation in patients with ischemic stroke. Stem Cells. 28:1099-106.
- Banerjee S, Bentley P, Hamady M, Marley S, Davis J, et al. (2014) Intra‐arterial immunoselected CD34+ stem cells for acute ischemic stroke. Stem cells translational medicine. 3(11):1322-30.
- Bhatia V, Gupta V, Khurana D, Sharma RR, Khandelwal N. (2018) Randomized assessment of the safety and efficacy of intra-arterial infusion of autologous stem cells in subacute ischemic stroke. Am J Neuroradiol. 39(5):899-904.
- Cepoi L, Donţu N, Şalaru V, Şalaru V. (2016) Removal of organic pollutants from wastewater by cyanobacteria. In Cyanobacteria for bioremediation of wastewaters Pp: 27-43, Springer, Cham.