The Effects of Stem Cells on Epilepsy
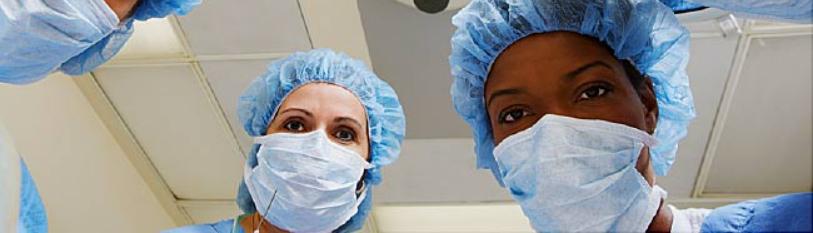
Alex N Hannegan1 and Vincent S Gallicchio2*
Department of Biological Sciences, College of Science, Clemson University, Clemson, South Carolina, USA
*Corresponding author: Vincent S Gallicchio, Department of Biological Sciences, College of Science, Clemson University, Clemson, South Carolina, USA
Citation: Hannegan AN, Gallicchio VS. (2021) The Effects of Stem Cells on Epilepsy. J Stem Cell Res. 2(1):1-19.
Received: December 18, 2020, | Published: December 30, 2020
Copyright© 2021 genesis pub by Hannegan AN, et al. CC BY-NC-ND 4.0 DEED. This is an open-access article distributed under the terms of the Creative Commons Attribution-Non Commercial-No Derivatives 4.0 International License., This allows others distribute, remix, tweak, and build upon the work, even commercially, as long as they credit the authors for the original creation.
DOI: https://doi.org/10.52793/JSCR.2020.2(1)-14
Abstract
Epilepsy is a neurological disorder that yields recurring and uncontrollable seizures, as a result of the hypersynchronous discharge of neurons that impacts individuals of all ages. Cases of epilepsy are classified by seizure type and etiology. Antiepileptic drugs (AEDs) are the standard treatment for the disorder, but surgical and neurostimulation options are also available; however, none of the mentioned treatments are 100% effective in eliminating seizures in epileptic patients. One-fifth of those diagnosed with epilepsy are AED resistant, also known as refractory epilepsy. Stem cell transplantation is a regenerative therapy capable of replacing non-functional cells in the brains of those with epilepsy. Embryonic stem cells (ESCs), mesenchymal stem cells (MSCs), neural stem cells (NSCs), and induced pluripotent stem cells (iPSCs) are capable of differentiating into specialized cell types. Stem cell therapy as an alternative treatment of epilepsy has exhibited success by way of preclinical animal research and clinical trials.
Keywords
Cellular therapy; Clinical trials; Epilepsy; Nervous system; Neurons; Seizures; Stem cells
Introduction
Epilepsy is a disorder of the central nervous system yielding unprovoked and recurrent seizures [1]. Seizures are a result of the uncontrollable hypersynchronous discharge of neurons in the brain [2]. On a fundamental level, seizures are prevented by maintaining an ionic environment with a resting membrane potential [2]. This resting membrane potential is typically set close enough to the firing threshold, so that neurons are able to undergo polarization at a rate that is not excessively high [2]. An excess discharge can be due to low amounts of Gamma aminobutyric acid (GABA) in the brain. GABA is the primary inhibitory neurotransmitter, as it limits the excitement of a wide range of neurons; when GABA is present in insignificant quantities, neurons have the ability to fire frequently without suppression [3].
There are three primary categorizations of seizures: focal (partial), generalized, and epileptic spasms [1]. Focal seizures are derived in just one hemisphere of the brain, while generalized seizures occur bilaterally [1]. The two have their own taxonomies, although it is possible for a seizure to begin focally and become generalized with the passage of time [1]. Focal seizures consist of simple partial and complex partial seizures; generalized seizures can be further classified into absence, tonic-clonic, myoclonic, and atonic seizures [1]. These classifications of seizures distinguish themselves by the characteristics of the individuals undergoing an epileptic attack [4]. For an example of a generalized seizure, an individual undergoing a myoclonic seizure will experience a sudden jerk in their extremities, potentially resulting in the person falling over [4]. On the other hand, an individual experiencing a complex partial seizure (focal) will likely have an altered awareness and become dazed [4]. Figure 1 exhibits the subcategories of partial and generalized seizures, providing common symptoms of the individual experiencing the seizure. Electroencephalograms (EEGs) and other neuroimaging machinery measure abnormal electrical activity occurring in various regions of the brain. These practices often give researchers intel on the location and intensity of seizures [1]. In Epileptic individuals, hyperventilation and photic stimulation help induce more epileptic activity, resulting in more drastic findings in neuroimaging [1].
Figure 1: Flow chart of seizure types and characteristic symptoms [5].
Epilepsy is one of the most common disorders of the brain, affecting approximately 65 million individuals worldwide [4]. It is estimated that 7.1 of every 1,000 people in the United States are diagnosed with epilepsy [6]. The incidence is found to be even higher in low-income countries [7]. Even with the burden of the disease seeing a decrease in recent years, people with epilepsy are at a higher risk of death than people without the disorder [7]. The disorder’s adjusted life years, a measure of the years lost living in those with the disease, was greater than 13 million globally [7]. The total number of sudden unexpected death in Epilepsy (SUDEP) is second in lost life-years among neurological cases; stroke was the only one higher [8]. Despite this, the majority of epileptic deaths are not due to SUDEP and seizure itself, but rather to resulting bodily actions [8]. Drowning, motor vehicle accidents, falls, burns and much more are examples of seizure related accidents that can lead to death [8].
There are multiple ways to classify epilepsies. Classification via etiology provides origins of the development of the epilepsy disorder [9]. The four major etiologies are idiopathic, symptomatic, provoked, and cryptogenic epilepsy, each with several subcategories [9].
Idiopathic epilepsy is an epilepsy disorder that is inherited and derived predominantly from genetics [9]. It is estimated that 70% of all epileptic individuals have idiopathic epilepsy [10]. Symptomatic epilepsy can be acquired in addition to being derived genetically; it is broken into two major subgroups: predominantly genetic or developmental causation and predominantly acquired causation [9]. Provoked epilepsy is described as epilepsy developed due to an external factor [11]. This designation is complex; for a provoked seizure to occur, both excitement and a predisposing cause must be present [9]. An analogy made, compared this to a spark and gunpowder-both are necessary for an action to occur [9]. Although an underlying cause and excitement are both needed, the amount in which the precipitate is responsible for the seizure has little effect in the intensity of the seizure itself [11]. Lastly, cryptogenic epilepsy has an undisclosed origin but is believed to be symptomatic [9]. As cryptogenic epilepsy can fall into the symptomatic etiological classification, along with provoked epilepsy, the remaining 30% of epileptic individuals fall into the symptomatic etiology [12]. (Table 1) provides, in detail, the subcategories and examples of the etiologies of epilepsies mentioned. Site of onset is an alternate method of classifying epilepsy; as more knowledge is made available about the disorder, more classification methods will likely surface.
Main category |
Subcategory |
Examples |
|
Idiopathic Epilepsy |
Pure epilepsies due to single gene disorders |
Benign familial neonatal convulsions |
|
Autosomal dominant nocturnal frontal lobe epilepsy |
|||
Severe myoclonic epilepsy of childhood |
|||
Pure epilepsies with complex inheritance |
Idiopathic generalized epilepsy |
||
Benign partial epilepsies of childhood |
|||
Symptomatic epilepsy |
Childhood epilepsy syndromes |
West syndrome |
|
Lennox-Gastaut syndrome |
|||
|
Predominately genetic or developmental causation
|
Progressive myoclonic epilepsies |
Unverricht-Lundborg disease |
Mitochondrial cytopathy |
|||
Neurocutaneous syndromes |
Tuberous sclerosis |
||
Neurofibromatosis |
|||
Disorders of chromosomal function |
Down syndrome |
||
Fragile X syndrome |
|||
Isodicentric chromosome 15 |
|||
Developmental anomalies of cerebral structure |
Hemimegaloencephaly |
||
Hippocampal sclerosis |
Hippocampal sclerosis |
||
Predominately acquired causation |
Prenatal and infantile causes |
Neonatal seizures |
|
Vaccination and immunization |
|||
Cerebral trauma |
Open head injury |
||
Closed head injury |
|||
Neurosurgery |
|||
Cerebral tumor |
Glioma |
||
Cerebral infection |
Viral/bacterial meningitis |
||
Cerebrovascular disorder |
Cerebral hemorrhage |
||
Degenerative vascular disease |
|||
Cerebral immunologic disorders |
Rasmussen encephalitis |
||
Degenerative and other neurologic conditions |
Alzheimer disease |
||
Multiple sclerosis and demyelinating disorders |
|||
Provoked epilepsy |
Provoking factors |
Drug/alcohol and toxin induces seizures |
|
Reflex epilepsies |
Photosensitive epilepsies |
||
Startle-induced epilepsies |
|||
Hot-water epilepsy |
|||
Cryptogenic epilepsy |
Unknown |
Account for 40% of adult epilepsies |
Table 1: Scheme for etiological classification of Epilepsy [9].
Treatment
Epilepsy is prevalent among people of all ages [13]. Individuals are diagnosed with the neurological disorder that is characterized by successive seizures, less than twenty-four hours apart from each other [13]. The second seizure is critical in the diagnosis of epilepsy; only one-third of children that experience a first-time seizure will eventually develop epilepsy [14]. In a large proportion of epilepsy cases, people receive video-EEGs to confirm seizure type and obtain an estimate of the epileptogenic zone [13]. There are several modalities of treatments for individuals diagnosed with epilepsy; antiepileptic drugs (AEDs), surgery, and neurostimulation have all served as viable options, with AEDs being the most customary option [14]. The ultimate goal of AEDs is to completely inhibit seizure activity in the patient’s brain, without introducing side effects; only 50% of AED treatments have accomplished this goal [15]. Cognitive side effects are the most common consequence of opting for pharmaceutical treatment; sedation, somnolence, distractibility, insomnia, dizziness, and an altered perception of quality of life are all examples of cognitive side effects patients may experience [16]. For example, the AED, Vigabatrin, underwent a troublesome process receiving approval from the Federal Drug Administration [14]. In 2010, it was officially approved, despite 30% of all patients experiencing irreversible bilateral concentric visual field constriction [14]. Children commonly experience aggression and hyperactivity as a side effect of AEDs, while adults experience depression with more regularity [16]. Although certain side effects vary among cohorts, the extremes of age experience side effects at a significantly higher rate [16].
Even with the possibility of side effects, today 70% of children are able to control their epileptic attacks with medication alone [14]. This is due in large part to the rise of new age AEDs. There are currently twenty-four distinguishable AEDs [14]. These new age AEDs have a smaller risk of side effects compared to previous AEDs [16]. AEDs are classified based on providing broad spectrum or narrow spectrum treatment, in addition to certain seizure specific AEDs [14]. (Table 2) shows each individual AED and its classification. The medication selected for treatment of epilepsy is dependent of several qualities of the patient, including the type of seizure experienced, age, gender, drug interactions, and cost [15]. In most patients, polytherapy is recommend over monotherapy, due to the implications of multiple therapeutics functioning in a simultaneous fashion; however, in pregnant woman, monotherapy is recommended [14]. The percentage of congenital malformation is higher among children with epileptic mothers, compared to infants with nonepileptic mothers [14]. There is evidence of a relationship reliant upon dosage between fetal exposure to valproic acid (VPA) and cognitive abilities in offspring [14]. For these reasons, pregnant mothers looking for epilepsy treatment typically undergo monotherapy in low dosage or seek an alternate method of treatment.
Broad Spectrum |
Narrow Spectrum |
Seizure Specific |
|
Clonazepam |
Carbamazepine |
Absence: Ethosuximide Valproic acid Lamotrigine |
|
Felbamate |
Ezogabine |
||
Lacosamidea |
Gabapentin |
||
Lamotrigine |
Oxcarbazepine |
||
Levetiracetama Rufinamide |
Perampenel Phenytoina |
||
Infantile spasms Adrenocorticotropic hormone Vigabatrin
|
|||
Topiramate |
Pregabalin |
||
Valproatea |
Tiagabine |
||
Zonisamide |
Vigabatrin |
Table 2: Classification of Antiepileptic Medications as Broad or Narrow Spectrum [14].
Treatment of epilepsy with AEDs may be the most common method of treatment, but under certain circumstances an alternate approach must be taken. It is estimated that one third of epilepsy patients experience recurrent seizures that are unable to be treated with AEDs alone[17]. A case of epilepsy is deemed drug resistant once two different tolerated AEDs are unsuccessful in controlling seizures [17]. The movement to surgery as an epilepsy treatment was due in large part to Victor Horsley; in the late 19th century, he removed scar tissue from the frontal lobe of a patient experiencing chronic seizures [18]. This resulted in the eradication of the patient’s seizures and paved the path for future surgical practices [18]. Temporal lobe epilepsy (TLE) is the most common form of epilepsy that is drug resistant by a wide margin [18]. There are multiple surgical options available to those with drug resistant TLE. Two common operations performed under these circumstances are anterior temporal lobectomy and selective amygdalohippocampectomy [18]. Corpus colostomy is an effective operation in epileptic individuals with generalized seizures [19]. Severing the corpus callosum prevents the two hemispheres of the brain from communicating with one another, making epileptic attacks less substantial [19]. While 62% of patient’s families, of those receiving a corpus colostomy, report improvement in daily functioning, the surgery completely inhibits seizures in less than 10% of patients [20]. Approximately one third of all individuals who opt for a surgical treatment of epilepsy see no improvements in controlling seizures, while another third experienced significant improvements but still required AEDs to further inhibit epileptic activity [21]. The last third of patients receiving surgery reported to be completely seizure free [21].
Another modality of epilepsy treatment is neurostimulation. Recent technological developments allow for treatment by delivering stimuli to a specific target site [22]. One method of neurostimulation is repetitive transcranial magnetic stimulation (rTMS) [22]. This delivers a magnetic wave up to 2 cm deep from the surface of the skull; this is deep enough for the magnetic wave to reach the cortex, providing treatment in this region of the brain [22]. Studies have illustrated that rTMS reduced seizure occurrence by 72% for periods greater than 2 months [22]. Another common neurostimulation technique is vagus nerve stimulation (VNS) [22]. VNS requires the implantation of a neurocybernetic prosthesis in the chest of an epileptic patient [22]. The device delivers electrical currents in the vagus nerve, triggering organelles in the brainstem and reducing seizure activity by 50% in the limbic system [22]. This deep brain stimulation is ideal when epileptic patients are classified as drug resistant, especially in those that are not surgical candidates [23].
Though various viable treatments exist, proper treatment techniques are not available in many regions of the world [24]. An estimated 4 in every 5 individuals that suffer from epilepsy live in middle or low income countries [24]. Analyzing the treatment gap, the number of individuals that need treatment but do not receive it, there are many more untreated epilepsy cases in countries with a low average income, compared to countries with a high average income [15]. The sizable treatment gap in low income countries is due to various factors, such as lack of knowledge, poverty, deficient health delivery infrastructure, and limited of professional health care workers [24]. It is estimated that the mortality rate of people with epilepsy is two to three times higher in low income areas than that of the entire population [24]. Advancements have been made in the last several years in every aspect of epilepsy treatment; with no treatment having been found to be completely successful thus far, it is important to look to stem cell therapy as a plausible treatment of epilepsy.
Stem Cells Therapy
Stem cells are those that possess the potential to differentiate into various different specialized cells [25]. These cells are found in organisms in the embryonic, fetal and adult stages of life [25]. Stem cells are classified in two ways, their ability to differentiate and their origins [25]. Classification based upon differentiation consists of totipotent, pluripotent, multipotent, oligopotent and unipotent cells [26]. Totipotent cells can differentiate to become any cell type and create an entire organism [26]. Pluripotent cells can form nearly all cells, differentiating into cells originating from the endoderm, mesoderm, and ectoderm, the three germ layers of the embryo [25]. Multipotent cells differentiate more narrowly into closely related cells [27]. Lastly, oligopotent and unipotent cells specialize into an even narrower selection of cells, with unipotent cells only producing cells of their own kind [26].
In addition to potency and differentiation, stem cells are categorized based on their origins. Embryonic stem cells (ESCs) are obtained from the pre-implantation period of the fertilized ovum in humans, commonly referred to as a blastocyst [28]. The blastocyst contains an inner-cell and outer-cell mass. The inner-cell mass is responsible for embryo formation; therefore, ESCs are harvested here [25]. ESCs are pluripotent and unique in the aspect that they can remain in their undifferentiated state for extended periods of time [25]. Although ESCs have numerous qualities that make them advantageous for potential clinical applications, obtaining the cells requires the destruction of a blastocyst, creating ethical roadblocks [28].
Adult stem cells (ASCs), also termed somatic stem cells, are totipotent and multipoint cells located in numerous regions of the body in the postnatal stage of life [27]. In recent years, findings regarding the plasticity of ASCs have been encouraging [29]. Tissue-resident stem cells have the ability to provide regenerative repair; the ectoderm is believed to give rise to skin and neural entities [29]. Mesenchymal stem cells (MSCs) are a customary ASC, which can be isolated from bone marrow, adipose tissue, umbilical cord blood, and amniotic fluid [25]. ASCs are vastly important to methods of stem cell therapy, as they can differentiate into many types of specialized cells, without creating ethical controversy [25].
A newer stem cell origin is known as induced pluripotent stem cells (iPSCs) [25]. These are ASCs that have been genetically modified to exhibit characteristics of ESCs [25]. This development has potential groundbreaking implications, considering cells could be harvested from human somatic cells, avoiding the ethical concerns that coincides with ESCs [30]. The genetic modification introduces the ability to differentiate in a pluripotent fashion, without causing any harm to a human blastocyst [30]. Technological developments are currently underway in order to make iPSCs a viable treatment in humans, but there are still many obstacles to overcome before it can become a regular clinical practice [30].
Embryonic Stem Cells
ESCs have many differentiation pathways, making them, ethics aside, an intriguing option for epilepsy treatment [31]. Despite this intrigue, several aspects of ESC therapy need to be improved before they can be applied clinically to neurological disorders [32]. Potential problems include the lack of control in proliferation; this has been best accomplished thus far by combining the methods of using feeder cells, supplementing growth factors and practicing genetic engineering [32]. Another potential problem in hESC involves the survival of stem cell derived neurons after transplantation and the adverse effects post-grafting [32]. Although it is relatively rare, a big concern is the formation of tumors at the site of grafting; tumors can be residual proliferating ESCs or precursor cells [32]. A study observed the tumor incidence in rats was reduced when the hESC were co-cultured for a period of 20 days [32]. Another recommendation for reducing the risk factor of tumors is to undergo drug-induced apoptosis of undifferentiated hESCs [32]. When health risks are successfully averted, post-grafting survival requires the avoidance of inflammation and graft rejection; these instances can be evaded with immunosuppression, the induction of immunotolerance, and somatic cell nuclear transfer [32].
Despite many risk factors associated with the direct use of ESCs, ESC-derived neural precursor cells have experienced promising preclinical animal trials [33]. In one study, ESC-derived neural precursors were obtained from the fetal human brain (ventricle zone) and delivered to epileptic mice; rats received the progenitor cells via injection in the tail [33]. The delivered cells were able to migrate to areas of the brain exhibiting seizure activity, including the hippocampus, piriform cortex, and the amygdala [33]. Results showed that nearly 26% of transplanted cells were GABA positive in the piriform cortex, and 31% were positive for Parvalbumin [33]. This provides hope for this method as a treatment of epilepsy, given that fully differentiated neurons lose their ability to divide and multiply [33]. Although encouraging, this study does provide difficult interpretations, due to no measurement of the number of excitatory neurons compared to GABAergic cells [33].
In a study conducted in 2009, researchers conducted a bilateral transplantation of precursor cells originating in the embryonic medial ganglionic eminence (MGE) to postnatal neocortex of mice [34]. This study allowed for additional evidence regarding the transplantation of cells as a treatment of epilepsy [34]. Significant results were observed in epileptic mice lacking a Shaker-like potassium channel (Kv1.1) [34]. The channel mentioned mimicked channel activity associated with human epilepsy disorders [34]. The Kv1.1 mice received either MGE grafts, or no treatment (control group) [34]. Results showed that mice receiving grafts developed GABAergic neurons, yielding significant reductions in both the length and regularity of spontaneous electrographic seizures [34] (Figure 2).
Figure 2: Seizure suppression in the Kv1.1 mouse model of Epilepsy [34].
A grade IV electrographic seizure was deemed to have frequency, synchronized high voltage, and polyspike or paroxysmal shape waves with amplitude >2-fold background, lasting more than 6 seconds [34]. (A) An EEG from a controlled Kv1.1 mouse during a grade IV epileptic seizure, and (B) the same seizure in higher resolution split into four different stages of the seizure progression, have a noticeably longer progression than that of (C) the EEG from the Kv1.1 mouse grafted with MGE [34]. The shorter progression of the seizure in the Kv1.1 mouse grafted with MGE is even more apparent with (D) the higher resolution EEG, split into four progression stages [34]. (E, F) The duration and recurrence of seizures was significantly greater in mice that were not grafted with MGE [34]. Lastly, the results indicate (G) the suppression of seizure activity in the grafted mice was less significant compared to the control Kv1.1 mouse [34]. This was seen, despite the probable overestimate in the number of seizures occurring in mice grafted with MGE, due to a longer period of monitoring for seizures [34]. The findings of this study advocate for the possibility of MGE interneuron precursor transplantation as a treatment for epilepsy in those lacking completely functional potassium channels [34]. Overall, the use of ESCs as treatment for epilepsy has too many risks to be accepted for clinical applications immediately; however, there are many promising preclinical findings associated with ESC derived neural precursor cells [32]. With continued research, the risk factors and ethical concerns involving ESCs may be reduced. But for now, and the immediate future, other stem cell therapies should be investigated for potential groundbreaking discoveries.
Mesenchymal Stem Cells
MSCs are multipotent and can be found in several regions of the body, including bone marrow, adipose tissue, umbilical cord blood, and amniotic fluid [25]. With even more areas of MSC isolation, many consider MSCs to be the most practical potential clinical application of stem cell therapy [25]. In a successful animal preclinical trial, conducted in 2017 by Salem et al., MSCs were isolated from bone marrow and delivered to male rats; this allowed for the application of MSC use as a treatment of TLE to be seen [35]. The MSCs were isolated from the bone marrow of three male rat’s femurs and tibiae; they were then suspended in 1% penicillin-streptomycin medium and incubated at 37° C for 7 days [35]. 4 x 103cells/cm2 were obtained and used for experimentation [35]. MSCs were labeled with PKH-26, a pink fluorescent die, allowing for easier discrimination among cells after transplantation [35]. With the use of a Hamilton syringe, 3mL of suspension was injected into both sides of the hippocampus (~100,000 cells on each side) [35]. In addition to this hippocampal bilateral injection, rodents were also injected intravenously, allowing for the two modes of stem cell delivery to be compared [35].
Results from this study provide evidence of the significant reduction of inflammatory cytokines, TNF-a and IL-1b [35]. Additionally, an improved oxidative state in the hippocampus was observed, due to antioxidant defense markers, GHS and PON1 [35]. Although it was thought that the intravenous method of injection would be more successful due to the direct access of lesioned areas, the bilateral hippocampal injection yielded double the cell count of the intravenous method [35] (Figure 3).
Figure 3: Photomicrograph of stained rat’s hippocampus at CA1 region (Scale bar of 100 mm) [35].
As seen in figure 3, in control rodents, (A) normal CA1 layers are observed with (B) an unharmed vesicular nucleus [35]. Meanwhile, (C, D) rodents with TLE exhibit focal axon degeneration and (C) accumulation of microglial cells [35]. (E) Rodents receiving bilateral hippocampal injection showed normal hippocampal structures, with benign perineural edema[35]. On the other hand, (F) rodents receiving intravenous method of injection revealed slight neuronal degenerative changes and perivascular edema [35].
In a similar MSC experimental design, rodents were randomly distributed into three groups: (1) control group with no procedure, (2) intravenous MSC delivery from bone marrow (1.0 x106 cells in 1 ml fresh DMEM, (3) intravenous vehicle injection (1 ml fresh DMEM) [36]. Seizures were administered using the Lithium-pilocarpine model; adult male rats were injected with lithium chloride (127 mg/kg in 0.9% NaCl) [36]. The next day, methylscopolamine bromide (1mg/kg in 0.9 % NaCl) was injected into the rats to limit peripheral effects of convulsions [36]. 30 minutes later, pilocarpine (20 mg/kg in 0.9% NaCl) was injected to provoke status epilepticus[36]. Seizures in the rats were observed via video-monitoring for 12 hours a day, 7:00 a.m. to 7:00 p.m., from day 21-30 [36]. Rats were then introduced to the Morris Water Maze Test and evaluated based on performance [36]. In the maze, rats were tasked with navigating a path to an escape platform with the use of visual cues placed around the perimeter of a submerged water tank [36]. All rodents were given the same maze pattern for 5 consecutive days, days 55-59 after the administration of Lithium-pilocarpine model [36].
Figure 4: Therapeutic effects of mesenchymal stem cells infusion in lithium-pilocarpine induced status epilepticus [36].
MSCs have also been conducted in human clinical trials as a treatment method of epilepsy [37]. In an experiment conducted by Hlebokazov et al., MSCs were derived from bone marrow [37]. The cells were cultured in a 5% incubator at 37° C in a modified Eagle’s medium[37]. Subjects receiving MSC treatment were required to be 18 years of age, diagnosed with epilepsy, considered to be refectory, no response to AEDs in the prior 2 years, and provided informed consent [37]. The patients were randomized into 2 experimental groups: (1) receiving standard treatment with AEDs (serving as the control group), or (2) receiving AEDs in addition to a single intravenous administration of undifferentiated MSCs (1 x 106 cells/kg), followed up by an intrathecal injection of neuro-induced MSCs (0.1 x 106 cells/kg) [37]. Two more patients revived the control therapy (n=12) than patients that received the cellular therapy (n=10) [37].
This study found that 70% of patients in the cellular therapy group experienced a shift from generalized tonic-clonic seizures to complex partial seizures [37]. 50% of the cellular therapy group also showed improved cognitive status [37]. The number of respondents by seizure frequency was also higher in the cellular therapy group (80%), than that of the control group (16.7%) [37]. Despite some promising results in the mini-mental state exam (MMSE), seizure frequency, seizure severity, anxiety, and seizure free remission of 6 months, the ability of MSCs to transdifferentiate into neural cell types remains debated [37]. Although certain conditions resulted in the over-production of neurotrophic factors (DNDF and NGF), some question the validity of differentiation from mesodermal origins, providing possible alternative explanations [37].
This study was performed without a placebo control and a lower than desirable number of patients [37]. Future human preclinical trials need to be conducted, with longer follow-up procedures and more patients [37]. Advancements in the identification of optimal survival routes and bioavailability, along with the ideal quantity of cell administration to achieve long-term therapeutic effects can be made [37]. Ultimately, there is encouraging data presented on seizure frequency data, leading to belief that MSCs can one day serve as a potential therapy for neurological disorders [37].
Neural Stem Cells
Neural Stem Cells (NCSs) are a promising tool to be used to when grafting in those with epilepsy because they can be cultured from several sources, including the fetal, postnatal and adult brain [38]. NSCs can synthesize glia secreting substances, which have anticonvulsant qualities, as well as GABA neurotransmitters [38]. NSCs are multipotent and believed to be capable of generating the major cell types of the CNS (astrocytes, neurons, and oligodendrocytes) [39]. In the adult brain neurogenesis is only located within two regions – the subventricular zone of the lateral ventricle and the subgranular zone of the hippocampus [40]. Once neurons have matured, they forfeit the ability to undergo cell division; cellular replacement via NSCs, is viewed as a potential treatment of neurodegenerative diseases [33].
In a rodent model, fetal neurons from a wide variety of brain regions were transplanted to the substantia nigra and hippocampus to measure seizure suppression ability [33]. These neurons resided in neuron-rich regions of the brain, the noradrenaline-rich locus coeruleus, the GABAergic-rich fetal striatum, and hippocampal regions [33]. It was found that these transplantations were successful in reducing abnormal electrical activity in the rodent brain [33]. Specifically, calbidin-immunoractive neuron cell death was significantly decreased [33]. Mossy fiber growth, the growth of granule cell axons into their own dendritic field, were prevented too [33]. With evidence of a reduction in recurring seizures in animal models in response to neural cell transplantation, NSC therapy as an epileptic therapy can be researched further [33].
In a study conducted by Chu et. al., NSCs were delivered to epileptic adult rats [41]. The NSCs were prepared from the ventricular zone of the embryonic human brain; in order to identify the cell transplanted, HB1.F3 cells were treated with replication-incompetent retroviral vector encoding, b galactosidase and puromycin-resistant genes [41]. Also, cerebrum cultures were treated with v-myc oncogene [41]. The rodents in the experiment underwent induced status epilepticus via lithium-chloride and pilocarpine injections [41]. Each rodent received an injection score, and a measure of the behavioral response to the saline of the injection [41]. After recurring seizures were induced, the adult rodents were randomly assigned to either an epilepsy only group, or NSCs transplanted group [41]. 28 to 35 days after treatment, the rat’s seizure activity was video-monitored for 12 hours each day, with each camera assigned to 3 rodents [41]. Seizures were scored using Racine’s scale, which classifies stages of seizures based on the subject’s actions [41]. Once the 6-week marker was reached, the animals were killed, and their brains were processed for histological purposes [41]. To identify transplanted cells, the hippocampal region of the brain underwent b gal immunofluorescent staining [41].
Treatment |
Seizure frequency (ictus/day) |
Rats developing seizures (%) |
Racine’s scale |
Injection score |
Epilepsy-only |
1.37 ± 0.22 |
85.1 ± 2.4 |
4.7 ± 0.3 |
4.2 ± 0.1 |
NSCs-transplanted |
0.05 ± 0.02 |
13.3 ± 1.8 |
4.2 ± 0.2 |
1.1 ± 0.3 |
Table 3: The effect of neural stem cell transplantation on spontaneous recurrent seizures [41].
Six weeks after transplantation, b gal+ cells were seen in the CA1, subiculum, hilus of dentate gyrus, CA3, amygdala and piriform cortex areas of the hippocampus [41]. The CA1 regions experienced the largest number of b gal+ cells, with 186.2 ± 26.4 cells/area discovered [41]. On the other hand, the thalamus and cerebellum had a miniscule amount detected (5 ± 4 cells/area) [41]. It was found that the long term neuronal damage of the NSC-transplant group was not statistically different than that of the epilepsy-only group [41]. Although this statistical relationship could not be determined in this study, it was positive that no tumor growth was detected in an intravenous method of delivering NCSs [41].
In a similar rodent model, the effect of NSC treatment on recurring seizures and efficacy was examined in rats with induced TLE [42]. In the procedure of the mentioned study, rats received a hippocampal NSC graft; results were compared to rodents receiving sham surgery (injections of culture medium, rather than NSCs graft) [42]. In the harvesting of NSCs, MGEs of E14 fetuses were dissected; MGE-NSCs were chosen for grafting because MGE is responsible for the most GABAergic interneurons in the fetal brain [42]. A week after treatments were administered, a significant reduction in frequency and intensity of epileptic activity was detected[42]. In rats receiving NSC grafts, an 82-90% reduction in all seizures were experienced, and an 89-93% reduction in stage-5 seizures was seen [42]. In the chronic stage of recurring seizures, the rodents receiving NSC grafts had a significant reduction in the amount of high amplitude spikes on EEGs [42]. It was seen that these rats had a 40-55% reduction of high amplitude spikes [42]. The same study provided information on the differentiation of grafted NSCs. The yield of graft-derived cells was approximately 28% (81,536 cells per hippocampus) of cells that were initially injected [42]. These graft-derived cells were capable of giving rise to mature neurons and other neural cellular entities [42]. The grafted MGE-NSCs differentiated into NeuN+ neurons, GABA+ interneurons, S-100b+ (a mature astrocyte), and NG2+ (an oligodendrocyte progenitor) [42]. Upwards of 10,000 neurons, 46,000 astrocytes, 2,000 oligodendrocytes progenitors, and 8,000 GABAergic neurons were developed in the hippocampus of several epileptic rats [42]. These measurements amount to the differentiation of over 40,000 new GDNF+ cells [42]. This is encouraging data, considering a vast amount of neurological components were produced.
In another study, the differentiation potential of NSCs was analyzed. NSC sample suspensions (200,000 cells/dish) were plated onto poly-D-lysine differentiation medium [43]. The cells were then incubated for a week at 37°C; the incubator was humidified and contained 5% CO2 [43]. Immunostaining was performed to categorize neuron differentiation into 4 categories: (1) those expressing beta-III tubulin (TuJ-1), (2) astrocytes expressing the glial fibrillary acidic protein (GFAP), (3) oligodendrocytes expressing protein O1, (4) GABA expressing interneurons [43]. A counterstain was also performed with DAPI, allowing for the nuclei to display a blue fluorescence when viewed under a microscope [43] (Figure 5).
Figure 5: Differentiation of plated NSCs after days 4 and 8 [43].
After 4 days of differentiation, it can be seen that Tuj-1, GABA O1 and GFAP all made a significant percentage of the total cells counted; O1 had the highest percentage, with approximately 15% of the total cells [43]. When differentiation reached day 8, all cell components increased in total percentage, compared to the percentages seen in day 4 [43]. O1 cells experienced the largest increase from day 4 to 8, accounting for over 50% of the cells by day 8 [43]. It is encouraging to see the ability of NSCs to differentiate in vitro [43].
Despite the great potential for NSCs to serve as an alternate treatment of epilepsy, there are some issues that must be resolved, prior to human clinical applications [44]. NSCs are found in specialized niches of the adult brain [44]. Neurogenesis occurs in the presence of angiogenic and astroglial niches of their own, which monitor self-renewal and differentiation of stem cells; therefore, it is vital that NSCs are able to complete their differentiation mechanisms in the niches in which they reside [44]. In addition to microenvironment selectivity issues, the culturing procedure of NSCs results in a heterogeneous sample of neural progenitor cells, limiting therapeutic effect [44]. Conducting additional research and animal models may yield advancements in the harvesting and controlled differentiation of NSCs, allowing them to be strongly considered as a treatment method in those with AED resistant epilepsies [44].
Induced Pluripotent Stem Cells
iPSCs are stem cells that have undergone genetic modifications to have pluripotent properties [30]. Neural and other brain related cell types have differentiated from iPSCs, including astrocytes, oligodendrocytes, and motor neurons [45]. iPSCs, if conducted safely and efficiently, are an optimal cellular therapy method because of their pluripotent properties, without the destruction of a blastocyst [30]. The concept of iPSC has transformed the concept of regenerative medicine because of personalized therapy potential; however, the transplantation of human iPSC-derived NSCs into the human brain is yet to be accomplished [45].
In a monkey model, the delivery of iPSC-derived NSCs in the monkey brain resulted in differentiation of neural cells without inflammation or tumor formation [45]. The successful grafting procedures in primates may be beneficial in future human trails [45]. Research has identified MGE as the superior source of interneuron progenitor formation; in rodent trials, hESC-derived neurons implanted in the hippocampus exhibited traits of GABAergic interneurons [45]. This grafting procedure was able to attenuate and completely eliminate seizure activity in mice [45]. These preclinical animal studies indicate why pluripotent cells are a desired method of treatment.
Like ESCs, minus the ethical concerns, iPSC treatment is not an issue of biomedicine, cell replacement therapy, pharmacology, or toxicology; issues reside in the safe delivery of iSPCs in vivo [46]. Safer delivery can be achieved through a direct differentiation method, minimizing the extent of mutations and transformation of injected cells [46]. In addition, cultivation of iSPCs without the use of animal cells is essential to avoid animal to human viral pathogen transfer.[46]. Lastly, the optimal conditions for iPSC cultivation and differentiation need to be solidified for maximum benefits to be experienced [46]. In recent studies, groups have bypassed the iPSC stage all together, by a method of direct programming; this method forces the expression of specific transcription factors, limiting the potential for tumorigenesis and unwanted differentiation [46]. Technological developments are currently underway to make iPSCs a viable treatment in humans, but there are still many obstacles to overcome before it can become a regular clinical practice [30].
Conclusion
Stem cell transplantation is an effective potential alternative in reducing the frequency and severity of the spontaneous and recurrent seizures associated with epilepsy. Although AEDs, surgical procedures and neurostimulation treatments are all viable treatments of epilepsy, many cases of epilepsy are AED resistant and there is no 100% effective treatment of the neurological disorder. For the reason, stem cells’ capacity of self-renewal and differentiation yield potential in replacing damaged and dysfunctional neurons. The pluripotent capabilities of embryonic stem cells make them promising treatment alternative, since they have potential to differentiate to any type of cell in the human body; however, due to risk of tumor development, improvements must be made before it is an accepted clinical treatment. Mesenchymal stem cells can be isolated from all three germ layers and differentiate in a multipotent fashion. The ability to differentiate into neurons is still questioned in prior research, so further preclinical and animal tests are recommended before mesenchymal stem cells can be viewed as a viable alternative. Neural stem cells are capable of producing many cell types found within the brain, specifically within the central nervous system. While easier access to these stem cells must be created, they offer promising future developments of the treatment of epilepsy. Lastly, induced pluripotent stem cells offer many similar qualities of embryonic stem cells, minus the major ethical barriers, making them a promising alternative if risk factors can be averted. Ultimately, more authorized human clinical trials need to be conducted in order to advance research on the topic of stem cells as a therapy for epilepsy, despite the great potential show thus far.
References
- Stafstrom CE, Carmant L. (2015) Seizures and epilepsy: An overview for neuroscientists. Cold Spring Harbor Perspectives in Medicine. 5(6):a022426.
- Scharfman HE. (2007) The neurobiology of epilepsy. Curr Neurol Neurosci Rep. 7(4):348-54.
- Gale K. (1992) GABA and epilepsy: Basic concepts from preclinical research. Epilepsia. 33(Suppl 5):3.
- Johnson EL. (2019) Seizures and epilepsy. Med Clin North Am. 103(2):309-24.
- Seizure Types & First Aid. Types of Seiz ures. (2020).
- Atlas: Epilepsy care in the world. World Health Organization. (2005).
- Beghi E. (2020) The epidemiology of epilepsy. Neuroepidemiology. 54(2):185-91.
- Devinsky O, Spruill T, Thurman D, Friedman D. (2016) Recognizing and preventing epilepsy-related mortality: A call for action. Neurology. 86(8):779-86.
- Shorvon SD. (2011) The etiologic classification of epilepsy. Epilepsia. 52(6):1052-7.
- Heron SE, Scheffer IE, Berkovic SF, Dibbens LM, Mulley JC. (2007) Channelopathies in idiopathic epilepsy. Neurotherapeutics. 4(2):295-304.
- Shorvon SD, Guerrini R, Andermann F. (2011) Introduction to the concept of provoked epilepsy. In: The causes of epilepsy. Cambridge University Press.
- Pitkanen A, Lukasiuk K. (2009) Molecular and cellular basis of epileptogenesis in symptomatic epilepsy. Epilepsy Behav. 14(Suppl 1):16-25.
- Guerreiro CA. (2016) Epilepsy: Is there hope? Indian J Med Res. 144(5):657-60.
- Sankaraneni R, Lachhwani D. (2015) Antiepileptic drugs--a review. Pediatr Ann. 44(2):36.
- Ali A. (2018) Global health: Epilepsy. Semin Neurol. 38(2):191-9.
- Ortinski P, Meador KJ. (2004) Cognitive side effects of antiepileptic drugs. Epilepsy & Behavior. 5:60-5.
- Miller JW, Hakimian S. (2013) Surgical treatment of epilepsy. Continuum (Minneap Minn). 19(3 Epilepsy):730-42.
- Asadi-Pooya AA, Rostami C. (2017) History of surgery for temporal lobe epilepsy. Epilepsy Behav. 70(Pt A):57-60.
- Spencer SS, Spencer DD, Williamson PD, Sass K, Novelly RA, et al. (1988) Corpus callosotomy for epilepsy. Neurol. 38(1):19.
- Asadi-Pooya AA, Sharan A, Nei M, Sperling MR. (2008) Corpus callosotomy. Epilepsy & Behavior. 13(2):271-8.
- Dasheiff RM. (1989) Epilepsy surgery: Is it an effective treatment? Ann Neurol. 25(5):506-9.
- Lin Y, Wang Y. (2017) Neurostimulation as a promising epilepsy therapy. Epilepsia Open. 2(4):371-87.
- DeGiorgio CM, Krahl SE. (2013) Neurostimulation for drug-resistant epilepsy. Continuum (Minneap Minn). 19(3 Epilepsy):743-55.
- Mbuba CK, Newton CR. (2009) Packages of care for epilepsy in low- and middle-income countries. PLoS Med.6(10):e1000162.
- Kolios G, Moodley Y. (2013) Introduction to stem cells and regenerative medicine. Respiration. 85(1):3-10.
- Abdelalim EM. (2016) Recent advances in stem cells. Cham: Springer International Publishing AG.
- Fortier LA. (2005) Stem cells: Classifications, controversies, and clinical applications. Vet. Surg. 34(5):415-23.
- Eguizabal C, Aran B, Chuva de Sousa Lopes SM, Geens M, Heindryckx B, et al. (2019) Two decades of embryonic stem cells: a historical overview. Hum Reprod Open. 2019(1):hoy024.
- Wagers AJ, Weissman IL. (2004) Plasticity of adult stem cells. Cell. 116(5):639-48.
- Braganca J, Lopes JA, Mendes-Silva L, Almeida Santos JM. (2019) Induced pluripotent stem cells, a giant leap for mankind therapeutic applications. World J Stem Cells. 11(7):421-30.
- Boison D. (2009) Engineered adenosine-releasing cells for epilepsy therapy: Human mesenchymal stem cells and human embryonic stem cells. Neurotherapeutics. 6(2):278-83.
- Li JY, Christophersen NS, Hall V, Soulet D, Brundin P. (2008) Critical issues of clinical human embryonic stem cell therapy for brain repair. Trends Neurosci. 31(3):146-53.
- Maisano X, Carpentino J, Becker S, Lanza R, Aaron G, et al. (2009) Embryonic stem cell-derived neural precursor grafts for treatment of temporal lobe epilepsy. Neurotherapeutics. 6(2):263-77.
- Baraban SC, Southwell DG, Estrada RC, Jones DL, Sebe JY, et al. (2009) Reduction of seizures by transplantation of cortical GABAergic interneuron precursors into Kv1.1 mutant mice. Proc Natl Acad Sci U S A. 106(36):15472-7.
- Salem NA, El-Shamarka M, Khadrawy Y, El-Shebiney S. (2018) New prospects of mesenchymal stem cells for ameliorating temporal lobe epilepsy. Inflammopharmacology. 26(4):963-72.
- Fukumura S, Sasaki M, Kataoka-Sasaki Y, Oka S, Nakazaki M, et al. (2018) Intravenous infusion of mesenchymal stem cells reduces epileptogenesis in a rat model of status epilepticus. Epilepsy Res. 141:56-63.
- Hlebokazov F, Dakukina T, Ihnatsenko S, Kosmacheva S, Potapnev M, et al. (2017) Treatment of refractory epilepsy patients with autologous mesenchymal stem cells reduces seizure frequency: An open label study. Adv Med Sci. 62(2):273-9.
- Shetty AK. (2010) Neural stem cell therapy for temporal lobe epilepsy. Epilepsia. 51:92.
- Al-Mayyahi RS, Sterio LD, Connolly JB, Adams CF, Al-Tumah WA, et al. (2018) A proteomic investigation into mechanisms underpinning corticosteroid effects on neural stem cells. Mol Cell Neurosci. 86:30-40.
- Thodeson DM, Brulet R, Hsieh J. (2018) Neural stem cells and epilepsy: Functional roles and disease-in-a-dish models. Cell Tissue Res. 371(1):47-54.
- Chu K, Kim M, Jung K, Jeon D, Lee S, et al. (2004) Human neural stem cell transplantation reduces spontaneous recurrent seizures following pilocarpine-induced status epilepticus in adult rats. Brain Res. 1023(2):213-21.
- Shetty AK. (2012) Neural stem cell therapy for temporal lobe epilepsy. In: Jasper's basic mechanisms of the epilepsies. Oxford University Press.
- Upadhya D, Hattiangady B, Shetty GA, Zanirati G, Kodali M, et al. (2016) Neural stem cell or human induced pluripotent stem cell-derived GABA-ergic progenitor cell grafting in an animal model of chronic temporal lobe epilepsy. Curr Protoc Stem Cell Biol. 38: (1):2D-7.
- Philippe Taupin. (2009) Adult neurogenesis, neural stem cells and Alzheimer’s disease: Developments, limitations, problems and promises. Curr Alzheimer Res. 6(6):461-70.
- Du X, Parent JM. (2015) Using patient-derived induced pluripotent stem cells to model and treat epilepsies. Curr Neurol Neurosci Rep. 15(10):71-3.
- Medvedev SP, Shevchenko AI, Zakian SM. (2010) Induced pluripotent stem cells: Problems and advantages when applying them in regenerative medicine. Acta Naturae. 2(2):18-28.sss