Stem Cell Therapies in Anterior Cruciate Ligament Reconstruction
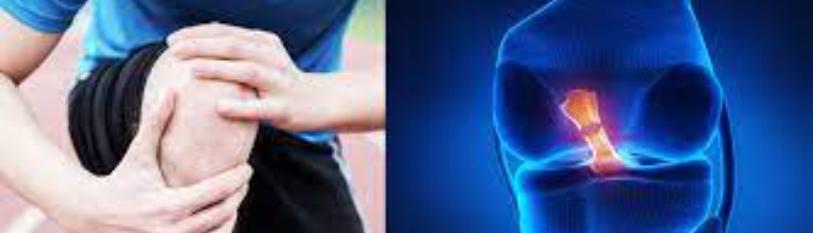
Mason R Pearce and Vincent S. Gallicchio*
Department of Biological Sciences, College of Science, Clemson University Clemson, SC 29636
*Corresponding author: Vincent S. Gallicchio, Department of Biological Sciences College of Science Clemson University Clemson, SC 29636
Citation: Pearce MR, Gallicchio VS. (2022) Stem Cell Therapies in Anterior Cruciate Ligament Reconstruction. J Stem Cell Res. 3(2):1-27.
Received: May 27, 2022 | Published: June 16, 2022
Copyright© 2022 genesis pub by Pearce MR, et al. CC BY NC-ND 4.0 DEED. This is an open-access article distributed under the terms of the Creative Commons Attribution-NonCommercial-No Derivatives 4.0 International License., This allows others distribute, remix, tweak, and build upon the work, even commercially, as long as they credit the authors for the original creation.
DOI: https://doi.org/10.52793/JSCR.2021.3(2)-35
Abstract
Anterior cruciate ligament (ACL) rupture is one of the most common knee injuries with an annual incident rate estimated to range between 30 to 78 per 100,000 person-years. To treat a ruptured ACL surgical reconstruction (ACLR) using a tendon graft is the current standard. While tendon grafts offer a viable solution to knee instability caused by ACL rupture many patients may face secondary pathologies like post traumatic osteoarthritis which can be devastating to the young, physically active population that this injury effects. These poor outcomes following ACLR may be associated with poor ACL graft healing and the unnatural, inferior scar tissue formation postoperatively at the tendon-bone enthesis. Stem cell therapies have been proposed not only as a therapeutic option for this substantial issue, but to also accelerate healing to decrease the lengthy time it takes to retain normal knee mechanical function. Many promising stem cells have been analyzed in ACL graft regeneration such as: bone marrow mesenchymal stem cells (bMSCs), ACL-derived stem cells, tendon derived stem cells (TDSCs), adipose derived stem cells (ADSCs), periosteum derived stem cells (PDCs), umbilical cord blood derived mesenchymal stem cells (UCB-MSCs) and induced pluripotent stem cells (iPSCs). Of the Multiple analyzed stem cell lineages many have demonstrated the ability to effectively both improve and accelerate ACL graft healing. This literature review will discuss and summarize the current knowledge, methods, limitations, and future prospect of stem cell therapies in ACL graft regeneration.
Keywords
Mesenchymal stem cells; Anterior cruciate ligament; Tendon graft; ACL reconstruction
Abbreviations
Anterior cruciate ligament: ACL; Anterior cruciate ligament reconstruction: ACLR; Bone-patellar tendon-bone: BPTB; Hamstring tendon: HST; Quadriceps tendon: QT; Bone marrow derived mesenchymal stem cells: bMSCs; Mesenchymal stem cells: MSCs; embryonic stem cells: ESCs; Extensor digitorum communis: EDC; Bone marrow cell: BMC; Semitendinosus tendon: ST; Extensor digitorum longi: EDL; Dedifferentiated osteogenic bMSCs: De-bMSCs; bone volume/total volume: BV/TV; plasma rich plasma: PRP; Stem-cell conditioned media: CM; demineralized bone matrix: DBM; Mesenchymal precursor cells: MPCs; Hyaluronan: HA; ligament progenitor/stem cells: LPSCs; flexor digitorum longus tendon: FDLT; Tendon derived stem cells: TDSCs; Bone mineral density: BMD; Muscle derived stem cells: MDSCs; Adipose derived stem cells: ADSCs; Synovial mesenchymal stem cells: SMSCs; Periosteum derived cells: PDCs; human umbilical cord blood-derived mesenchymal stem cells: hUCB-MSCs; Induced pluripotent stem cells: iPSCs; Bone marrow mononuclear cells: BMMNCs; Extracellular vesicles: EVs
Introduction
Stem cells are unspecialized cells present in multicellular organisms that can differentiate into many specialized cell lineages. Given the properties of stem cells much attention has been drawn to them for research and novel medical application to treat a multitude of diseases and injuries. Specifically, the idea of stem cell therapy in restorative and regenerative medicine has been of focus and has a tremendous amount of potential. An injury that has significant positive possibilities through treatment with stem cell therapies is anterior cruciate ligament (ACL) rupture/tear. The ACL is a primary ligament in the tibiofemoral joint and ranges from 27 to 38 mm in length and 854 to 1,854 mm3 in volume. The volume of the ACL primarily consists of highly aligned collagen fibers that are separated into two bundles: anteromedial and posterolateral [1]. The ligament attaches to the lateral and anterior aspects of the medial intercondylar spine on the tibia and to the medial aspect of the lateral femoral condyle [2].
Rupture of the ACL is one of the most common and devastating knee injuries with an annual incident rate estimated to range between 30 to 78 per 100,000 person-years [3-9]. The ACL plays a primary role in preventing anterior tibial translation relative to the femur as well as a secondary role in internal and external rotational restraint [2]. Injury/tear of the ACL is commonly associated with mechanisms that place significant strain or force on the ligament like a quick cutting, deceleration, jumping, or pivoting movement commonly seen in sports which is why younger individuals, athletes, or people participating in sports/physical activity are the primary affected population by this injury [3]. The mechanism of ACL injury can be either contact or non-contact. Among ACL injuries around 70% of patients report a non-contact mechanism or a 7:3 ratio of non-contact to contact mechanism [10,11].
An estimated 100,000 anterior cruciate ligament reconstruction (ACLR) procedures are performed each year in the United States making it one of the most common sports medicine orthopedic procedures [12]. There are multiple variations/protocols for ACLR that have been developed, but among them autografts (graft being used is from patients own tissue) are the gold-standard with a much lower failure rate compared to allografts (tissue taken from another human) [13-19]. Among ACLR autograft procedures the bone-patellar tendon-bone (BPTB) autograft, hamstring tendon (HST) autograft, and quadriceps tendon (QT) autograft are primarily utilized. HST and BPTB autografts are the most prominently used methods with minimal differences between the two methods noted [20,21]. Among athletes who have ACLR 83%-89% return to pre-injury sport with most patients reporting initial satisfactory outcomes after surgery [22-28]. Despite being a viable solution and providing patients initial stability ACLR has multiple postoperative problems.
The issues with ACL rupture begin with its poor healing capacity and regenerative capabilities. These factors not only lead to the necessity for surgical reconstruction rather than suturing or non-surgical approach but can be associated with secondary pathology after ACLR. Native anatomy of ACL entheses is a gradient: transitioning from dense fibrous connective tissue to uncalcified fibrocartilage to calcified fibrocartilage to periosteum [29]. The literature has shown that after ACLR instead of the ACL graft regenerating native tissue, it forms fibrovascular scar tissue at the graft-bone tunnel insertion that is less functional and inferior to a natural insertion point [30]. Significant attention has been placed on proper tendon-bone healing after ACLR as it is crucial for the best patient outcomes, yet it remains a problem after ACLR. This fibrous scar tissue formation and different microanatomy of the knee after ACL rupture can lead to changes from natural kinematic movement and knee positioning which are associated with secondary pathology like post traumatic osteoarthritis (PTOA) [31-34]. Following ACLR patients are at higher risk for ACL injury in bilateral knees, but especially in the contralateral ACL compared to healthy individuals of the same age in the same physical activity [35-37]. Tunnel widening/expansion is another gradual onset postoperative process associated with ACLR especially in HST grafts and allografts that may also be associated with knee instability [38,39]. Patients who have ACLR have been noted to have higher risk for osteoarthritis up to 13% if only the ACL is involved, however this number can be as high as 48% when associated with meniscal injury [40]. Development of osteoarthritis and other secondary pathology discussed above in the young, active population that ACL ruptures affect can be life altering and debilitating [3]. Another concerning statistic is that of the athletes that undergo ACLR only 45%-63% will return to pre-injury level of performance [27,28,41-44]. While this large percentage of failure to return to pre-injury level cannot be exclusively attributed to the change in knee kinematics and inferior tissue formation it is concerning that poor graft healing may have such a significant impact on athletic careers and patient’s lives. Additionally, patients must also consider time spent recovering and rehabilitating following ACL reconstruction as it can be a strenuous and long process. There is no set amount of time before the individual may be able to return to the prior physical activity as the amount of rehabilitation time can vary due to differential progress of knee stability among patients. Currently literature suggests individuals can experience deficits in the knee that underwent surgery anywhere from 6 months to 24 months following surgical reconstruction [45]. Meaning at the bear minimum an athlete/patient must miss at least half a year of sports or normal physical activity for rehabilitation to restore knee stability which can be devastating to one’s career and lifestyle.
Despite much progress with ACLR many post-operative problems persist that can chronically and significantly impact patient’s lives. Stem cell therapy has been proposed as a possible solution to these problems as it may accelerate and improve ACL graft healing to imitate native ACL anatomy. Therapeutic application of stem cells may allow an increased number of patients to return to activity faster, participate at pre-injury level, and decrease the postoperative pathology. The purpose of this scientific review is to provide a concise and comprehensive summary and discussion of the current research on possible stem cell therapies that could be utilized as a solution to problems associated with poor ACL regeneration after reconstruction.
Bone marrow derived mesenchymal stem cells (bMSC)
Mesenchymal stem cells (MSCs) are one of two types of non-embryonic stem cells (non-ESCs). MSCs are self-renewing, multipotent stromal cells that can be isolated from multiple different sources of tissue in the human body and proliferate extensively. MSCs are the primary stem cell used in ACL tissue regenerative research as they can differentiate into multiple related lineages or exclusively differentiated in to adipocytic, chondrocytic, and osteocytic lineages [46]. Stem cells derived from bone marrow are known as bone marrow derived mesenchymal stem cells (bMSCs). Given bone marrows composition it has historically been used to isolate MSCs, thus bMSCs are the most extensively used stem cell to study stem cell enhanced healing after ACLR.
One of the first uses of MSCs in ACL regenerative research was in 2004 when bMSCs were transplant via mixing in fibrin glue which was coated on the HST autografts at the time of reconstruction on adult rabbits [47]. The study found that treated groups had formation of fibrocartilage that resembled that of a native enthesis and at 8 weeks had significantly higher failure load and stiffness than control groups that were not treated with bMSCs therefore indicating better osteointegration. Soon et. al., [48] used Achilles’ allografts with very similar superior experimental group results in biomechanical strength and tendon-bone interface histology resembling a normal ACL insertion. Other non-ACL model studies focused on tendon-bone healing have demonstrated similar positive results in the ability of bMSCs to enhance tendon-bone healing [49-51]. Babb et. al., [52] examined the use of bMSCs in skeletally immature rabbits undergoing ACLR utilizing extensor digitorum communis (EDC) autograft. This study found that the experimental group including bMSCs had no bone bridge formation, no growth arrest, and no angular deformity which are common problems observed in pediatric ACL repair cases. Chen et. al., [53] used a very similar technique to the previously mentioned studies to understand the properties of gene and protein expression caused by bMSCs during tendon-bone healing after ACLR. The study demonstrated enhanced native-appearing fibrocartilage formation in bMSC-fibrin glue group through promotion of type II collagen and aggrecan expression as well as reduction of Runx2 and osteocalcin expression causing overall chondrogenesis. Two studies, Oe et. al., [54] and Kanaya et. al., [55], examined ACL healing/regeneration after intraarticular injection of bMSCs in partially transected ACLs in rat models. Oe et. al., [54] found partially transected ACLs treated with bMSCs had superior tensile strength, no transected area retraction, higher collagen scores, and increased expression of TGF-P1 compared to that of the saline injection group. Kanaya et. al., [55] had similar results showing significantly better histological scores, no transected area retraction, and higher ultimate failure load at four weeks post-op compared to control group. Both studies concluded bMSCs enhanced healing in a partially ruptured ACL. A more recent study demonstrated decellularized allogenic semitendinosus tendon (ST) grafts in combination with injection of autologous bMSCs into bone tunnels were superior to fresh frozen allogenic graft alone in ligamentization, load capacity, and ossification [56]. Also recent, Hur et. al., [57] analyzed the effects of bMSCs on bone tunnel widening after ACLR using autologous extensor digitorum longi (EDL) tendons in a rabbit model. They found not only decreased tunnel widening in the bMSCs treated group but the bMSC treated group had better graft architecture, hypercellularity, and compact collagen deposition.
Kanazawa et. al., [58] had somewhat contrary findings when they utilized autologous bMSCs with an ST autograft ACLR model in which no bone tunnels were made. Only one specimen of seven from the bMSC group showed formation of a chondroid cell layer at both anterior and posterior interfaces with the study finding no significant differences between the bMSC group and control group. While the study concluded tendon graft healing was not enhanced by bMSCs it may be an indication of the importance of bone tunnels in ACLR surgical protocol and healing process of the ACL graft.
Overall, BMSCs have shown to be an effective stem cell at enhancing graft healing, however obtaining bMSCs is invasive and low numbers of them exist naturally in the bone marrow. Use of bMSCs alone may not be sufficient in ACL graft regeneration therefore researchers are looking at ways to further enhance differentiation and proliferation bMSCs as well as alternative stem cells that are less invasive to obtain.
Dedifferentiated osteogenic bone marrow derived mesenchymal stem cells (De-bMSCs)
Dedifferentiation is notable across natural biology and refers to the transformation of a cell from a more differentiated state to a less differentiated state where the cell can proliferate to regenerate tissue [59]. De-bMSCs are bMSCs that during the osteogenic differentiation had induction conditions removed and replaced with basal media (dedifferentiation). While much is still largely unknown about de-bMSCs they are an attractive candidate for stem cell transplantation in ACLR as they have exhibited improved osteogenic potential [60]. In 2021 Tie et. al., [61] utilized De-bMSCs transplanted in bone tunnels using alginate gel during autologous ST graft ACLR in a rabbit model. In comparison to bMSCs the De-bMSCs had significantly higher maximum failure load, stiffness, new bone formation around graft, and bone volume/total volume (BV/TV) values suggesting enhanced tendon-bone healing. Additionally, through measuring mRNA and protein expression levels it showed that the Nanog/NFATc1/Osterix pathway plays important role in enhancing osteogenic differentiation ability. This year (2022), Tie et al., [62] used an identical protocol but this time focused attention on biomechanical, micro-CT analysis, histological findings in comparison to bMSCs. Results showed: at 12 weeks de-bMSCs were found to have higher maximum failure load, stiffness, BV/TV value, smaller tunnel area, increased expression of osteoblast genes, and better histological appearance compared to bMSCs. These studies are an indication that De-bMSCs may be a superior option for stem cell therapies than untreated, normal bMSCs in enhancing ACL graft healing.
The results of these two studies are promising, however De-bMSCs are novel and little research has been conducted on their use. More studies are necessary to continue to understand their mechanism and effectiveness in ACLR graft healing.
Growth factors and bMSCs
Growth factors are important biochemical cues that direct the proliferation, differentiation, and migration of stem cells in regenerating tissue. Studies have demonstrated growth factor delivery alone can enhance ACL tissue healing [63-66]. This makes growth factors a promising possible choice for a combination therapy with stem cells to further enhance the activity of transplanted stem cells on ACL graft healing after ACLR. A popular choice for the delivery of growth factors is platelet-rich plasma (PRP). PRP is an autologous source of multiple growth factors like PDGF, EGF, TGF, IGF, FGF, and VEGF. In Teng et. al., [67] to analyze the effectiveness of growth factors in combination with stem cells the study transplanted PRP with bMSCs via fibrin glue on autologous ST grafts in a rabbit model undergoing ACLR. The study had superior results in the PRP with bMSC group. The group showed increased osteogenic gene expression, better histological observations, increased mineralized tissue formation, and greater failure load at 8 weeks. The study concluded PRP stimulated osteogenesis in bMSCs enhancing tendon-bone healing. A very recent 2020 study, Hexter et. al., [68], also utilized combination delivery of PRP and bMSCs with important differences in model. The study examined a large animal model using sheep. Additionally, both graft and bMSCs were allogenic. The study supported results from Teng et. al., [67] as the PRP/bMSC group’s ACL grafts had a more native ACL appearance and superior maturation at 12 weeks pointing to enhanced ACL graft healing. These findings have possible future clinical importance as current therapeutic use of PRP has shown to be unsuccessful by itself in improving graft maturation and bone tunnel healing [69]. Setiawati et. al., [70] investigated combined delivery of VEGF and bMSCs also using fibrin glue in a rabbit model. Treated groups had better tensile strength, gradual MRI signal increase, and more collagen type III development than untreated groups indicating enhanced graft tunnel healing when treated with VEGF and bMSCs. Stem cell-conditioned medium (CM) is a novel, intermediate between stem cell transplantation and growth factor injection as it has less safety concerns due to being acellular yet possibly yielding similar benefits to growth factors and stem cells. Sun et. al., [71] utilized CM from allogenic bMSCs in a rat model undergoing ACLR and demonstrated that the CM was able to enhance bone-graft healing in vivo as well as stimulate -SMA+ myofibroblasts in vitro.
Another promising biologically derived material that contains multiple growth factors and has osteoinductive properties is demineralized bone matrix (DBM). A comparative analysis on growth factors and stem cells published by Hexter et. al., [72] in 2021 looked at three separate treatment groups on the same model as their prior study [68]. Allogenic tendon grafts were treated with DBM, PRP, and bMSCs. While the study concluded no treatment group significantly reduced bone tunnel widening it did indicate that when compared to other independent treatment groups DMB was significantly better at enhancing femoral bone healing in a large animal model using contemporary ACLR.
Delivery of growth factors seems to be a reliable short-term option to further enhance the functions of bMSCs, however there are important notable limitations with growth factors. Growth factors have shown to have a dose- and time-dependent effect meaning that for long term enhancement of bMSCs using growth factors they would need to be applied multiple times post-operatively [73]. This can increase site morbidity, be financially unfeasible, and be uncomfortable for the patient.
Bioengineering and bMSCs
There is growing interest in biologically engineered tissue grafts for a regenerative approach to ACL rupture and to reduce problems associated with ACLR. Biocompatible grafts are of interest as they may be able to provide the same or more biomechanical stability and strength associated with normal ACLR graft as well as enhance and accelerate ligament regeneration. Bioengineered tissues and scaffolds also are attractive for possible combination therapy with stem cells as they have shown to work well incorporating MSCs and provide a way of delivery of the cells [74]. In Fan et. al., [75] a bioengineered scaffold graft was constructed utilizing a silk mesh to imitate extracellular matrix and was seeded with bMSCs. In vitro analysis of the graft demonstrated gradual increases of bMSC proliferation and collagen deposition over the 2 weeks. In vivo analysis of the scaffold/bMSC treated group showed a more native ACL with direct bone insertion, hypercellularity, superior biomechanical strength, and abundant production of ligament tissue and collagen compared to the control group (scaffold without bMSCs). 1 year later Fan et. al., [76] utilized the same scaffold seeded with bMSCs, but this time on a swine model. Findings in this study supported those from the prior study [75] in that bMSCs enhance biological regeneration of ligament tissue, however they noted there was formation of indirect insertions at the entheses. Bi et. al., [77] designed a similar study to Fan et al. [75] with the exception that the bioengineered silk mesh scaffold graft was integrated with a type 1 collagen matrix (a major connective tissue in the ACL) from pigs Achilles’ tendons prior to being seeded with bMSCs. The results were positive with the bMSC seeded collagen scaffold group showing more significant integration of trabecular bone with graft at 16 weeks, increased bone mineral density, fibroblast-like cells, and tenascin-C expression. A major negative contrast to the other studies discussed however was that there was no more significant amount biomechanical strength noted in the treated group. An earlier 2012 study also used a type 1 collagen scaffold on a rabbit model but sutured the ruptured ACL rather than performing a full ACLR with graft [78]. The type 1 collagen-silk scaffold was sutured in on the ACL with the same sutures to correct the transected ACL. The study had overall more negative results as only 33% of the bMSC/collagen-scaffold treated rabbits showed they had regenerated ACL tissue and native appearing ACLs with histology consistent with natural ACL. Additionally, this study is more limited as it lacked biomechanical and microCT analysis of the ACL in comparison to the other studies. Li et. al., [79] utilized a triphasic-silk scaffold graft seeded with osteoblasts, chondrocytes, and bMSCs into appropriate regions to match that of a native ACL in a rabbit ACLR model. Triphasic scaffolds provide a unique, novel approach with bioengineered scaffolding grafts as a triphasic graft more closely simulates the tissue organization of a natural ACL compared to that of an isotropic scaffold used in prior studies. In vitro analysis found vast equal proliferation, differentiation, and native ACL tissue gene expression among the three lineages. In vivo the treated group had significant ligament-like regeneration, better osseointegration showing stratified layers in native ACL bone interface with increased mineralization, and superior biomechanical strength like that of a normal ACL. The researchers concluded that triphasic scaffolds with trilineage cells enhance both ACL regeneration and osseointegration increasing the functionality of the graft more than isotropic grafts with just bMSCs alone. Fan et. al., [80] also utilized a triphasic scaffold graft seeded with bMSCs but lentiviral vectors encoding growth factors TGFß3, and BMP were added to area B (fibrocartilage) and C (bone) of the three distinct areas of the scaffold. Examining the scaffold graft, three distinct areas exhibited differential cell types suggesting gene modification was successful in inducing proper cell lineage differentiation. Furthermore, the treated group had a bone ligament interface with stratified tissue layers, higher BV/TV ratio, and markedly more mechanical strength than control without genes. This study further confirms the conclusions about triphasic scaffolds from previous [79] but this shows an appealing approach as it offers an alternative, more efficient way of creating stratified tissue layers without the individual input of the three cell types. An electrospun nanofibrous scaffold is another new bioengineered scaffold type that has shown promise in regenerative science through its significant surface area, mechanism for gene delivery, and ability to adhere stem cells. Zhu et. al., [81] examined the use of an electrospun nanofibrous scaffold seeded with bMSCs and covalent bonding of MSC affinity peptide E7. The scaffolds were braided to HT autografts on a rabbit model undergoing ACLR. The study found that bMSCs expressing the gene had no bone tunnel enlargement with gradual decrease in diameter at 3 months, superior biomechanical strength, native ACL tissue formation, and gradual transition at enthesis.
These studies have shown that bioengineered scaffolds in combination stem cells are an appealing alternative to current tissue grafts and may be a superior way to transplant stem cells. Scaffolds seeded with bMSCs have shown to successfully regenerate ACL tissue and facilitate proper healing at enthesis. In the small amount of time that bioengineered tissues have been utilized in combination with MSCs much progress has been made. Early studies have been even further improved in both histological as well as biomechanical aspects with the fabrication of more advanced scaffolding and inclusion of biological agents to enhance bMSCs [79-81]. Bioengineered scaffolds in combination with bMSCs is a promising field of research and with its continued innovation this has very realistic application in regenerative medicine.
Gene therapy bMSCs
Use of cytokines and growth factors to enhance the functionality, differentiation, and proliferation of bMSCs in vivo has shown to be effective through combination exogenous delivery with the cells as mentioned in previous sections. A barrier associated with growth factors is that these positive effects are a short-term success due to their short half-life. To circumvent this issue researchers have looked to gene-therapy to not only extend the time-dependent effects of these growth factors but offer an improved approach to achieve better ACL regeneration. Li et. al., [82] was first to utilize gene therapy with autologous bMSCs for in vivo ACL regeneration. Their study transfected the human PDGF-B gene into retroviruses which were then used to transduce the gene into the bMSCs. Rabbits underwent ACLR using an irradiated Achilles allograft that was coated in the PDGF-B gene transduced bMSCs using fibrin glue. In the bMSC + PDGF-B group early hypervascularity, increased collagen deposition, and hypercellularity was recognized. By 12 weeks both the bMSC group and bMSC + PDGF-FF had native appearing ACLs more so in gene transduced group. A study with closely similar methods was conducted by Dong et. al., [83] examining bMSCs transduced with BMP-2 via lentiviral gene carrier. Their study showed that bMSCs + BMP-2 had superior biomechanical strength, stiffness, and more fibrocartilage tissue formation at 8 weeks compared to bMSCs that were not transduced. Their research overall indicated that BMP-2 may be an effective agent to enhance bMSCs function in tendon-bone healing. Chen et. al., [84] further evaluated BMP-2 by transplanting bMSCs transduced with bFGF and BMP-2 genes in ACLR using a rabbit model. The study found the BMP-2/bFGF expressing bMSC group showed a broader area of cartilage-like cells, substantial higher maximum load, and better stiffness. Their findings point to co-expression of BMP-2/bFGF being a better option to enhance bMSCs in ACLR than BMP-2 or bFGF alone. Wei et. al., [85] also used bMSCs that were co-expressing growth factor genes. The study examined VEGF and TGFß-1 expression in bMSCs that were transplanted via fibrin glue in rabbits undergoing ACLR with an Achilles allograft. Their study indicated that the VEGF/ TGFß-1 group had significantly more biomechanical strength at 24 weeks when compared to growth factors transduced alone and was able to promote angiogenesis at 3, 6, and 12 weeks. The study demonstrates that co-expression has advantageous, synergistic consequences compared to single growth factor expression in enhancing ACL graft healing. Wang et. al., [86] further elaborated on the function of TGFß in transduced bMSC on tendon-bone healing through either inhibiting or overexpressing TGFß in the bMSCs experimental groups. Their study utilized rabbits undergoing autograft ACLR with bMSC transplanted via injection into tendon-bone tunnel and joint cavity. The results of this showed significantly more tendon-bone healing at 12 weeks, increased mechanical load, hypercellularity, and MAPK signaling proteins in the over-expression group when compared to the control and under-expressing group. Additionally, it was shown that the group treated with TGFß under-expressing bMSCs had significantly worse ACL graft healing pointing out the importance of TGFß in the ACL graft healing process. They concluded TGFß over-expression improves ACL graft healing through impacting the MAPK pathway which further supports previous results [85]. A recent 2018 study employed a novel method for gene therapy by using ultrasound guided BMP-6 gene delivery to endogenous MSCs in a large animal (porcine) model with allograft ACLR [87]. The study concluded that the genes delivered with ultrasound were effectively expressed on intraarticular endogenous stem cells and had significantly better healing capabilities of the ACL graft in comparison to that of the group without ultrasound mediated delivery.
Gene therapy as an approach to better the capabilities of mesenchymal stem cells in improving ACL graft and tendon-bone healing seems to be promising. Overall, the literature is reporting superior functionality of the stem cells when transduced with the growth factor genes. Furthermore, co-expression of these genes in stem cells rather than single expression seems to be an even more innovative and successful method in improving ACLR due to its synergistic effects. Despite positive pre-clinical trials, the prospect of clinical trials is still distant given the novelty of this approach and unknown negative effects associated with viral guided gene alteration. Studies like have begun looking for alternative methods to deliver these genes to decrease possible factors like oncogenicity associated with gene therapy, but these methods are still in their infancy [87].
Clinical use of bMSCs
Despite the overwhelmingly positive results from the numerous pre-clinical studies on small animals that have been conducted to examine ACLR enhanced healing with bMSCs there are few large animal trials and even fewer clinical trials. Findings from the 4 clinical trials are inconsistent but are beginning to show some promise. Silva et. al., [88] was the first to utilize non-cultivated bMSCs on a human model and showed disappointing findings. In their study 43 patients underwent single-bundle ACLR with double-looped ST and gracilis grafts with 20 of the patients receiving bMSC injections. They evaluated patients at the 3-month post-operative stage via MRI and histological analysis. Their study concluded that non-cultivated bMSCs do not accelerate ACL tendon-bone healing. This study was followed a year later by Centeno et. al., [89] which had somewhat promising results. In this small case study autologous bone marrow concentrate rich in mesenchymal stem cells was injected into patients with all three grades of ACL sprains without undergoing ACLR. The study found that the MRI imaging measures all decreased toward low-signal intensity and most patients had improved ACL integrity with objective and subjective measures. This study shows some promise for MSCs in human ACL healing but is limited by its lack of a control group and randomization. Wang et. al., [90] conducted a controlled, randomized study that followed patients for 2 years following a single injection of allogenic mesenchymal precursor cells (MPC) in hyaluronan (HA) after unilateral HST autograft ACLR. The 11 patients in the MPC + HA group demonstrated statistically significantly improvements in clinical scoring and radiographic assessments compared to control indicating allogenic MPCs in HA have no significant immune reaction and may be able to positively impact the development and symptoms of PTOA. A recent 2021 study investigating bMSC injection into patients undergoing allogenic BPTB also analyzed MRI imaging and subjective scoring throughout a 2-year post-operative period [91]. The study concluded bMSC can be clinically beneficial in early ligamentization after showing that patients treated with bMSCs had significantly better early radiograph signal intensity ratios and subjective outcome. The future direction of clinical use of bMSCs should continue to be researched given the inconsistent findings at this point.
Ligament derived stem cells
When compared to bMSCs ligament progenitor/stem cells (LPSCs) have identifiable advantages: tissue-specific derivation, greater proliferative potential, and higher expression of tendinous repair genes [92,93]. ACL derived CD34+ vascular stem cells were recently discovered and isolated by Matsumoto et. al., [94] in damaged ACL tissue. They identified that the ACL derived CD34+ vascular stem cells have multilineage differentiation potential, rapid proliferation capabilities, and were found in higher concentrations at injured ACL tissue which indicated the cells were possibly involved in ACL healing. Given that ruptured ACL tissue is abundant in CD34+ vascular stem cells autologous ruptured ACL tissue was utilized for transplantation in a canine model undergoing ACLR with an autograft [94,95]. The study concluded that ruptured ACL tissue can enhance early bone-tendon healing with significant increases in angiogenesis, osteogenesis, and biomechanical strength. Mifune et. al., [96] was the first notable study to utilize isolated cultured ACL derived CD34+ stem cells in a small animal model (rat) undergoing ACLR. Rats treated via intraarticular injection with the ACL derived CD34+ stem cells exhibited significantly better tendon osteointegration, angiogenesis, osteogenesis, bone-graft interface mass, VEGF levels, and biomechanical strength compared to CD34- group or control group. This study is an indication that isolated ACL derived CD34+ stem cells alone can enhance tendon-bone healing in an animal model. A year later, Mifune et. al., [97] combined isolated ACL derived CD34+ stem cells with bioengineering by culturing the stem cells to create cell sheets which were wrapped around the flexor digitorum longus tendon (FDLT) autografts in a rat model. The study compared injection versus cell sheet delivery of the ACL derived CD34+ stem cells and concluded that when delivered by cell sheet there was significantly better cellular incorporation, graft healing, and tendon-bone healing. The study indicated that bioengineered cell sheet mediated delivery is a superior method compared to intraarticular injection of the ACL derived CD34+ vascular cells for enhanced ACL graft healing. Hu et. al., [98] combined a bioengineered silk-collagen scaffold graft and intraarticular injection of isolated ACL derived LPSCs 1 week postoperatively in rabbit model. After evaluating at 3- and 6-months post-op they concluded that the injected ACL derived stem cells enhanced ACL healing. Ruan et. al., [99] similarly utilized a bioengineered knitted silk-collagen scaffold graft in a rabbit, however the LPSCs were cultured to form a cell sheet and wrapped on bioengineered graft. They utilized LPSCs derived from allogenic rabbit ACLs to investigate in vitro and in vivo. The study demonstrated that LPSCs have multilineage differentiation potential and cells incorporated well in the graft in vitro. In vivo analysis at 6 months postoperative revealed that the scaffold graft treated with LPSCs had significantly more collagen fibril formation, direct natural appearing ligament-bone incorporation, decreased joint space narrowing, and undamaged meniscus when compared to those that were not treated. The study concluded knitted silk-collagen graft wrapped with LPSCs can greatly enhance ACL healing and delay major secondary pathology associated with ACLR. To investigate the of role of growth factors and potential for gene therapy in LPSCs. Takayama et. al., [100] conducted a study examining ACL derived CD34+ cells virally transduced with either VEGF or sFLT-1 (antagonist to VEGF). The study used a rat model undergoing ALCR with autograft in which cells were transduced in vitro and then transplanted on the graft by wrapping the graft with a cell sheet. Their study was able to demonstrate that VEGF is a key growth factor in neo-angiogenesis and ACL healing. Moderate overexpression of VEGF in ACL derived CD34+ cells showed to accelerate graft healing compared to non-transduced ACL derived CD34+ cells. A year later Kawakami et. al., [101] used an identical model however ACL derived CD34+ cells were virally transduced to express BMP2. In vitro analysis of the cells demonstrated that the CD34+ cells expressing BMP2 were able to maintain angiogenic potential associated with vascular stem cells while having significantly more osteogenic differentiation compared to CD34+ cells not expressing BMP2. Evaluation of the nude rats treated with 25% expressing BMP2 CD34+ cells at 8 weeks revealed significantly higher tensile load-to-failure strength than all other groups as well as superior tendon-graft integration evidenced by immunohistochemical and histological analysis. This study further supports previous findings that CD34+ cells are effective at enhancing ACL healing, but further indicates that appropriate expression of BMP2 can accelerate tendon-bone healing. To evaluate when ACL derived CD34+ vascular cells are acquired from ruptured ligament tissue to have the best applicable healing efficacy two studies were conducted: Nakano et. al., [102] and Inokuchi et. al., [103]. To understand the age-dependent healing potential of ACL derived CD34+ cells either young (ages 10-19) or adult patients (ages 30-39) at mean time of 3.5 months post-rupture had cells removed [102]. After applying these cells to a rat model undergoing ACLR it was determined through biomechanical, morphometric, microCT, immunostaining, and histological analysis that ACL derived cells have an age-dependent efficacy on enhancing bone-tendon healing. In Inokuchi et. al., [103] the influence of time interval from rupture at which the ACL derived cells were obtained on healing potential was investigated. Cells were derived from early phase ruptured tissue as well as tissue from chronically ruptured ACL patients and applied to a rat model undergoing ACLR. After objective assessment it was found early phase derived ACL CD34+ cells have superior ACL graft healing effects compared to late stage. They concluded that ACL CD34+ cells have a time interval-dependent efficacy in enhancing tendon-bone healing.
Despite ACL derived stem cells being a relatively new discovery the current pre-clinical literature on ACL derived stem cells is promising for therapeutic application in enhancing human ACL graft regeneration. Limitations such as lack of evidence for fibroblast differentiation, low cell numbers, and surgical procedure to acquire cells are noted. Research on these cells should be a key focus in stem cell assisted ACL regeneration given the unique advantageous compared to other stem cell therapies [104] as well as consistent positive in vivo findings thus far. The future direction of ACL derived stem cells should be implemented to large animals and clinical trials to substantiate this potential.
Tendon derived stem cells (TDSCs)
Literature on TDSCs in ACL graft regeneration and tendon-bone integration is scarce. Lui et. al., [105] is the only notable study at this point to conduct pre-clinical research using TDSCs in ACLR. A rat model undergoing ACLR with a FDLT autograft was used with TDSC transplanted via a cell sheet delivery method. Biomechanical analysis of rats treated with TDSCs revealed significantly improved ultimate load and stiffness at weeks 2 and 6. The histology demonstrated that the TDSC group had better overall early tunnel graft integration and finer midsubstance findings at 6 and 12 weeks. Higher BV/TV and bone mineral density (BMD) were also exhibited in TDSC treated group. From these findings they concluded that TDSCs enhance early graft healing and tendon-bone integration. The origin of stem cells can have effects on their proliferation and specific differentiation therefore this makes TDSCs an attractive choice for musculoskeletal tissue regeneration [104]. TDSCs have demonstrated better proliferation, clonogenicity, tenogenic potential, chondrogenic potential, adipogenic potential, and osteogenic potential than bMSCs [106]. When compared with LPSCs, TDSCs lacked as much multilineage differentiation potential and have low cellularity when deriving them [107]. Much more research is necessary for determining the efficacy of TDSCs in ACL regeneration and should be strongly considered given their possible advantageous they present.
Muscle derived stem cells (MDSCs)
Literature on MDSCs in ligament/tendon regeneration is limited and has yet to be extensively evaluated. Sun et. al., [108] demonstrated, in vivo, that by leaving an appropriate amount of muscle tissue on the tendon grafts in ACLR there is more graft healing and incorporation than a tendon only graft. This shows potential for incorporating MDSCs in ACLR. In vitro investigation of MDSCs shows that the cells have osteogenic potential and can contribute to greater early ossification than TDSCs [109]. This shows utility and potential for MDSCs in enhancing ACLR. Additional research should be conducted to understand the role and application of these cells in ligament/tendon healing.
Adipose derived stem cells (ADSCs)
ADSCs have gained attention for wide application in research and clinical approaches due to their abundance and easy accessibility. This is contrary to most stem cells which necessitate invasive surgical approaches, secondary surgery, or cellular expansion to obtain meaningful cultures. ADSCs have been documented to be like bMSCs in multipotency, cellular characteristics, and proliferative efficiency [110,111]. In 2014 autologous ADSCs were first applied in vivo to ACL tendon graft regeneration utilizing a large animal model undergoing ACLR with a collagen scaffold [112]. The study had disappointing findings showing that neither peripheral blood MSCs or ADSCs provided a functional improvement at 15 weeks. In Kosaka et. al., [113] ADSCs were transplanted via fibrin glue in a rabbit model undergoing ACLR with an ST graft. Better early biomechanical and bone histological results were observed in rabbits treated with ADSCs which the study concluded delivered ADSCs enhance initial tendon-bone incorporation. Matsumoto et. al., [114] had similar findings indicating improved early biomechanical strength and tendon-bone healing. In their study they also used a rabbit model undergoing ACLR with ST autograft. To transplant the ADSCs they were cultured to form cell sheets and prior to graft insertion into the tibiofemoral joint the sheets were wrapped around the autograft. Ultimate failure load and histological scoring was observed to be statistically better at 2 and 4 weeks while stiffness showed to only be improved at week 2. Micro CT revealed less cross-sectional area in the femoral tunnel at 4 weeks but otherwise had no other significant early findings. Teuschl et. al., [115] investigated macroscopic and histological measures of ADSCs seeded on a silk fiber scaffold used in ACLR on a large animal model. Results demonstrated that the group treated with ADSCs seeded on the scaffold had decreased silk fibers and greater tissue regeneration at 6 months compared to scaffold alone, but no significant differences were noted at 12 months. The study indicates early ACL tissue regeneration may be expedited with ADSCs but yields no long-term enhancement in tissue arrangement. Three years later, Teuschl et. al., [116] revisited this exact model and methodology however with an emphasis on evaluating the osteointegration using microCT and bone histology. The study noted that the bioenhanced scaffold was able to initiate osteointegration but differences in bone tunnels between the ADSC seeded scaffold group and silk-scaffold alone group were minimal at 6 and 12 months. To investigate the potential of gene therapy in ADSCs in ACL regenerative research a study virally transduced Runx2 into ADSCs to be overexpressed [117]. In vitro analysis of the cells showed they had osteoblast differentiation while adipogenic differentiation was inhibited. In vivo analysis in a rat model was able to confirm ectopic bone formation as well as demonstrate significantly better bone tunnel ossification, better ultimate load failure up to 12 weeks, and direct attachment appearance at the enthesis by 12 weeks. Kouroupis et. al., [118] conducted a study utilizing human ADSCs (hADSCs) for in vitro fabrication of a bioartificial graft and in vivo application in an ACLR swine model. A group of hADSCs were cultured in TGF-ß/FGF-2 as well as a separate group of hADSCs in BMP-2/FGF-2. To selectively induce tissue formation the TGF-ß/FGF-2 group was seeded into the center of the biomaterial while the BMP-2/FGF-2 was seeded to the ends of the graft. Analysis of the bioartificial graft in vivo revealed triphasic tissue formation at the midsubstance and direct insertion at the enthesis. Overall, the findings concluded that the treated hADSCs cultured with biomaterial to form a bioartificial graft was able create a natural appearing ACL in vivo. Parry et. al., [119] has further demonstrated ADSCs are able to be successfully incorporated with biomaterial in vitro for possible in vivo delivery.
ADSCs are an attractive, practical option for clinical application given their therapeutic potential with small cost associated with acquiring them. Studies thus far have shown both positive and disappointing findings of their use in vivo. Given that ADSCs favor an adipogenic pathway of proliferation and differentiation it may limit the extent of their potential in tendon, ligament, and bone tissue regeneration.
Clinical use of ADSC
At this point only one clinical trial exists using ADSCs in ACLR by Alentorn-Geli et. al., [120] in 2019. The study analyzed 20 athletes undergoing ACLR using a BPTB autograft in which 5 mL of ADSC solution was delivered intraoperatively into the graft. The treated patients were compared to a historical cohort of 20 similar patients. Across 12 months the ADSC treated group had no significant difference based on MRI imaging and clinical scoring (VAS, IKDC, Tenger activity scale, Lysholm, and Lequesne) to control cohort. The study concluded that the addition of ADSCs intraoperatively did not enhance or provide any significant overall improvement to ACL healing. Research on ADSCs in ACL graft regeneration is inconsistent with studies reporting no enhancement or no long-term benefit. With the addition of gene therapy and more innovative biomaterials there is promise. Future studies should focus on the implementation of these methods to increase the functionality and potential of ADSCs as they continue to be a practical choice for application.
Synovial mesenchymal stem cells (SMSCs)
With the recent discovery of SMSCs they have gained increasing attention for musculoskeletal regeneration. They have shown to have multilineage differentiation capabilities such a chondrogenesis, adipogenesis, myogenesis, and osteogenesis [121,122]. Specifically, they have shown superior chondrogenic differentiation compared to cells derived from other tissues as well as higher proliferation rates in human serum than bMSCs [121-123]. Thus far, no studies have been conducted to specifically assess SMSCs in ACL regeneration. SMSCs have been evaluated in tendon-bone healing by Ju et. al., [124]. In their study they utilized a rat model that had an autologous Achilles tendon surgically placed in tibial bone tunnel with SMSCs injected into tunnel intraoperatively. The study found that at 1 and 2 weeks the treated group had a significantly better tendon-bone interface histology while at 4 weeks there were no observable differences. The study indicated that SMCs are effective in enhancing early tendon-bone healing. Going forward SMSCs should be implemented in an ACLR model in vivo to directly understand the efficacy or limitations they may have in ACL tissue regeneration.
Periosteum derived cells (PDCs)
The periosteum plays a key role fracture repair and in the musculoskeletal system as all tendons, ligaments, and muscle attach to it therefore cells derived from this layer of bone have become an attractive choice to enhance tendon-bone healing. It has been demonstrated that the periosteum contains cells with mesenchymal multipotency regardless of donor-age and higher proliferation than bMSCs [125,126]. Specifically, PDCs have shown superior osteogenic differentiation and calcification potential when compared to other stem cell derivatives [122,127]. PDCs have shown the ability to enhance tendon-bone incorporation in vivo with superior biomechanical strength and more natural enthesis histology [128,129]. PDCs were first applied clinically in 2004 to 62 patients undergoing ACLR using an autologous double loop ST and gracilis tendon graft who were then followed 2 years postoperatively [130]. In the study the experimental group had their grafts wrapped with uncultured periosteum taken from the proximal tibia around both ends. At final assessment data showed that that 79% of patients were able to return strenuous activity, 86% had full extension, a 94 median Lysholm knee score, and 92% reported normal knee function. Overall clinical assessment showed significant improvement from preoperative stage to postoperative stage with 81% assessed as normal with IKDC criteria. Chen et. al., [131], using the same methods again, analyzed 368 patients with at least 2 years follow up and were able to obtain similar findings. After conducting both studies [130,131] they indicated that periosteum treated grafts had satisfactory outcomes and may minimize bone tunnel enlargement. Both clinical trials are limited as they lacked a control, had small groups, and were not randomized. PDCs demonstrate clinical potential and are practical for application given that they can be easily derived during primary surgery. In the future isolated and cultured PDCs should be applied to an animal model specifically undergoing ACLR with graft as it has not yet been examined. Clinical research should focus on larger, randomized, and controlled methods to evaluate therapeutic efficacy.
Human umbilical cord blood-derived mesenchymal stem cells (hUCB-MSCs)
hUCB-MSCs are popular across stem cell research as they are already widely used in regenerative medicine. hUCM-MSCs are both ethically and non-invasively acquired and can be cryopreserved for later use [132]. Studies have demonstrated that hUCM-MSCs have low immunogenicity, multilineage differentiation potential, high proliferation, and superior collagen production [133-136]. This makes hUCM-MSCs an attractive candidate in ACL regenerative therapy. The literature on hUCM-MSCs in ACL regeneration is small. The first study to investigate the in vivo use of hUCM-MSCs was by Jang et. al., [137]. They utilized a rabbit model undergoing ACLR with a HST graft in which hUCM-MSCs were transplanted via fibrin glue into graft-tunnel interface. Histological assessment and tunnel scores at 4, 8, and 12 weeks showed superior results in the treated group with entheses at the 12 weeks resembling that of a native ACL which was not seen in control. MicroCT revealed smaller tunnel areas at later stages compared to control group, however tunnel size changes were not significant with time. It was concluded allogenic hUCM-MSCs can be applied without immune rejection and can enhance ACL healing. Later in 2018, hUCM-MSCs were applied via seeding on a three-dimensional scaffold sleeve and had similar findings [138]. Histological analysis of the treated group showed a more distinct, natural transition at the tendon-graft interface while immunohistochemical staining indicated substantially more type II collagen at all weeks in the treated group. At 12 weeks the microCT showed smaller areas for both the femoral and tibial tunnel. The study also concluded that hUCM-MSCs can improve ACL healing and osteointegration. Going forward pre-clinical studies with hUCM-MSCs should assess biomechanical strength as well as further combine the cells with other biological-enhancing factors to further promote regenerative properties of hUCM-MSCs.
Clinical use of hUCB-MSCs
Only recently in 2021 were hUCM-MSCs first applied clinically to ACL regeneration by Moon et. al., [139]. The study was double-blind and randomized looking at 30 patients in a positive control, negative control, and experimental group with minimal 2-year follow-up. The experimental group received intraoperative injection of allogenic hUCM-MSCs with HA into the graft-bone interface. The treated group showed no significant difference in tunnel volume measurements at 12 months, clinical parameters at 2 years, and second-look arthroscopy. The researchers concluded that while allogenic hUCM-MSCs caused no immune reaction they provided no advantage in clinical outcome or tunnel enlargement. This study may have been limited by the small sample size. While clinical use of hUCM-MSCs have disappointing findings thus far research should be conducted with a larger sample size and combine their use with other bio-enhancing factors.
ESCs & induced pluripotent stem cells (iPSCs)
ESCs are pluripotent cells that can differentiate to any non-extraembryonic cell lineage; however, use of these cells is severely limited in the clinical setting due their ethical and legal dilemma they present. To bypass this ethical dilemma iPSCs are used. iPSCs are cells derived from somatic cells that are induced in vitro to a pluripotent state like that of an ESC [140]. Given the diverse range of tissues that iPSCs can form and modern bioprinting techniques it makes them attractive for application in tissue regenerative therapies. In the previously mentioned study by Kouroupis et. al., [118] hiPSCs were also seeded on bioartificial grafts and applied to a swine model. The study’s findings showed that the group treated with hiPSCs had both morphological and biochemical characteristics that are exhibited by the native ACL. The study concluded that the hiPSCs can enhance ACL healing and possibly prevent secondary pathology like PTOA when compared to the control. Research using iPSCs in ACL regeneration is promising but is small. Going forward more in vivo studies with larger samples and different methods should be conducted using iPSCs to further understand the positive and negative effects they have in ACL regeneration.
Other cells and methods
Transplantation of bone marrow mononuclear cells (BMMNCs) have gained attention for tissue regeneration as they can be isolated from bone marrow in a short amount of time and do not require expansion unlike other stem cells. BMMNCs is an umbrella term that encompasses a wide variety of cells as it contains all the cells in the bone marrow that are mononucleated. BMMNCs have been used to treat various diseases including tissue ischemia with the intention of tissue regeneration [141-143]. Recently, BMMNCs were used in a rabbit model undergoing ACLR with a decellularized HST allograft in which cells were injected into the knee joint [144]. The study compared BMMNCs to a bMSC group and control group finding that BMMNCs caused an early immune reaction with relatively limited benefits to ACL healing. Although, the study indicated that the BMMNCs had comparable effects to bMSCs. Given the easy, low-cost clinical application of these cells further research should be explored to understand their success in ligament tissue regeneration.
Another method that has shown success in promoting tendon-bone healing are exosomes or extracellular vesicles (EVs). EVs are important for MSCs biogenesis and modulate/signal tissue regeneration in MSCs [145,146]. Two recent in vivo studies were conducted and demonstrated that bMSC derived EVs were able to promote tendon-bone healing and modulate cell action [147,148]. While EVs have not yet been utilized in an ACL model their use is promising and should be applied to ACL regeneration in future studies.
Conclusion
ACLR using a tendon graft is currently the gold standard solution for ACL rupture, however multiple problems present after ACLR. Stems cells offer a way to possibly bridge this gap by creating a more native ACL and reducing secondary pathology associated with ACLR like PTOA, contralateral rupture, and graft failure [35,40]. Almost all stem cells that were evaluated above were effective in enhancing ACL regeneration or improving tendon-bone healing to a certain degree. Introducing these stem cells in combination with gene therapy, bioengineered products, and stimulating factors further increase the functionality of these stem cells to regenerate native-like ACL tissue. Novel, innovative techniques of transplantation like cell sheets and bioengineered scaffolds ensure cell survival and increase proliferation compared to simple injection. Most of the current research regarding stem cells on enhancing ACL regeneration is on animal models and has shown great clinical prospect. Currently, of the few clinical trials most have failed to demonstrate the same substantial effects witnessed in vivo with studies just recently beginning to show somewhat similar positive effects. This lack of strong clinical evidence thus far is likely due to the relative infancy of the field of stem cell therapies as a whole and the small number of cell lineages and methods that have been applied clinically at this point. Notable limitations must be recognized and further understood such as clinical safety, immunogenicity, oncogenicity, invasiveness, surgical cost, and culture expansion. MSCs derived from ligament and tendon tissue have yet to be applied clinically to ACL regeneration but are very promising for future use. Very few studies exist comparing the different cell lineages and methods in enhancing ACL graft healing in vivo and should be a goal to conduct in the time ahead. Studies going forward must also address the mechanism by which these cells enhance ACL healing as well as focus on conducting more clinical studies with the other cell lineages and techniques that have been conducted in vivo.
Acknowledgement
I would like to thank Dr. Vincent S. Gallicchio for enabling and helping me to conduct this scientific literature review.
References