Application of Mesenchymal Stem Cells for the Treatment of Traumatic Brain Injury and Neurodegenerative Diseases
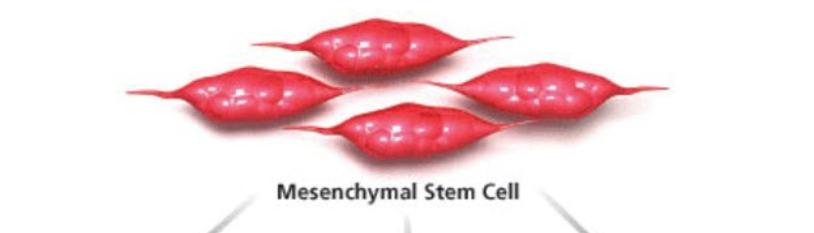
Kennedy Jordahl and Vincent S. Gallicchio*
Kennedy Jordahl and Vincent S. Gallicchio*
Department of Biological Sciences, College of Science, Clemson University, Clemson, South Carolina, USA
*Corresponding author: Vincent S Gallicchio, Department of Biological Sciences, College of Science, Clemson University, Clemson, South Carolina, USA
Citation: Jordahl K, Gallicchio VS. (2021) Application of Mesenchymal Stem Cells for The Treatment of Traumatic Brain Injury And Neurodegenerative Diseases. J Stem Cell Res. 2(1):1-21.
Received: February 12, 2021 | Published: March 03, 2021
Copyright© 2021 genesis pub by Jordahl K, et al. CC BY-NC-ND 4.0 DEED. This is an open-access article distributed under the terms of the Creative Commons Attribution-Non Commercial-No Derivatives 4.0 International License., This allows others distribute, remix, tweak, and build upon the work, even commercially, as long as they credit the authors for the original creation.
DOI: https://doi.org/10.52793/JSCR.2021.2(1)-17
Abstract
Despite the prevalence of traumatic brain injuries (TBIs) and neurodegenerative diseases, there is still a lack of effective and efficient therapeutic treatment options. TBI triggers an innate immune response and releases inflammatory molecules, creating a hostile environment that inhibits repair and regeneration. TBI has also been linked to a higher risk of suffering from neurodegenerative diseases, such as Parkinson’s, Alzheimer’s and Huntington’s disease in later years. Novel stem cell research has provided a treatment option that overcomes existing barriers and can be used in regenerative medicine. Mesenchymal stem cells (MSC) are of particular interest due to their easy obtainability, homing potentials, multipotent differentiation, and immunomodulatory aptitudes. The challenges of this cell therapy and future prospects are discussed as well. This review aims to comprehensively study the potential of mesenchymal stem cells in regenerative medicine for treatment of traumatic brain injuries and neurodegenerative diseases.
Keywords
Mesenchymal stem cell; Traumatic brain injury (TBI); Neurodegenerative disease; Neurogenesis; Neurons; Central nervous system; Treatment
Introduction
Traumatic brain injury (TBI) is one of the leading causes of death worldwide, and is a major contributor to long-term disability [1]. According to the Center for Disease Control and Prevention, approximately 1.7 million people suffer from TBI each year in the United States [2]. While 80% of TBIs are considered mild head injuries, moderate to severe TBIs are primary causes of induced death and disability. Adolescents aged 15 to 19 years old and older adults aged 65 years or older are the most likely to maintain a TBI and progression of a neurodegenerative disease. In recent years, the intensive care and management of TBI and neurodegenerative disease patients have significantly improved [3]. However, even under the best circumstances, there is 36% mortality for acute TBI; 15% severe disability; 20% moderate disability; and 25% complete recovery [4].
TBIs are inflicted via external sources. These external sources can include direct incidences such as collision, crash, or jolt to the head; or indirect incidences such as whiplash. In both cases, the normal structure and function of the brain is disrupted. Following the trauma inflicted on the brain, a cascade of events occurs, this includes the primary injury and a secondary injury consisting of several pathophysiological mechanisms. The duality of the human body’s innate inflammatory response to injury has protective and regenerative properties, yet also, destructive and inhibiting properties that make healing more difficult. This inability to regenerate neurons can be the onset neurological disease as well as inhibit pre-existing neurological impairment from being treated. The continuation of the body’s deleterious inflammatory response to TBI has been linked to neurodegenerative diseases. Neurodegenerative diseases are characterized by the loss of functional neurons in a debilitating and progressive manner, which can affect memory and physical capabilities. In fact, if neuronal death caused by TBIs continues to progress, the patient may be at higher risk of suffering from neurodegenerative disease in later years, such as Parkinson’s, Alzheimer’s, and Huntington’s disease [5-7]. The loss of neuron functionality caused by brain injury was previously thought to be irreversible [8,9]. Encouragingly, however, the current view is that the central nervous system consists of a variety of mechanisms allowing recovery. In fact, neurogenesis and angiogenesis in humans have confirmed to occur in a few areas of the brain.
While the primary impact of brain injury cannot be reversed, several leading stem cell therapy options may be useful in preventing secondary injuries and neurodegeneration. Of particular interest is the use of mesenchymal stem cells (MSCs) due to their ease of isolation, multipotent differentiation capabilities, tissue repair, homing potentials, ability to cross the blood-brain barrier (BBB), and immunomodulatory properties. Arguably the most important property of MSCs is their secretome. Trophic factors secreted by MSCs such as cytokines and growth factors, as well as neural protein expression, are predominantly responsible for the beneficial effects of this cell therapy [10]. While MSCs can be found in almost all adult tissues types throughout the body [11], MSCs from adipose tissue [12,13], bone marrow, and umbilical cord tissue [14] have shown great success in neural differentiation. All of these aspects make MSCs a promising prospect in regenerative medicine. Overall, this research review aims to comprehensively study the properties of MSC’s and their potential applications in regenerative medicine for treatment of TBIs and neurodegenerative diseases.
Discussion
Pathophysiology of TBI
Traumatic brain injury (TBI) is caused by external forces such as a jolt or blow to the head. The effects of TBI can be separated into external and internal consequences. Externally, the skull which protects the brain could be damaged; potential outcomes are hematoma, hemorrhagic contusion, herniation, and midline shifts of the brain. Internally, the complex BBB may be compromised by allowing immune cells to migrate into the central nervous system [15]. Normally, the BBB balances ion concentrations (Ca++, Na+, and K+), regulates what flows in and out of the brain, as well as protecting against foreign elements circulating in the bloodstream.
The primary stage is the initial, physical injury to the neurons, glial cells, nerve fibers, and the BBB [16,17]. This initial impact can cause local inflammation that exacerbates damage by spreading inflammation to surrounding neurons, ischemia (reduction of oxygen and glucose supply to cells), anaerobic respiration, lactic acid accumulation, loss of functioning ion pumps, and several cytotoxic effects; these consequences lead to further neuronal death [18-20]. The secondary stage initiates another cascade of events resulting in enlarged astrocytes that creates a barrier that surrounds the injured area. This physical barrier is called the glial scar which has both beneficial and inhibitory effects. This barrier protects the neurons still intact by isolating the site of injury and preventing inflammation from spreading to surrounding areas of the brain. However, this isolation slows the rate of macrophages entering the damaged area, prevents the regrowth of neurons, and inhibits the repair of the BBB and full recovery of functionality [21-23].
There is ongoing research aiming to find ways to reduce neurological damage and detrimental inflammatory processes, but a strong focus has been on the use of MSCs. It is also important to understand that the severity of TBI can affect the patient’s inflammatory response and thus treatment options. The severity and mechanism of injury is assessed to determine the extent of injury. Specifically, the Glasgow Coma Scale, a test ranging from 3-15 points, is used to assess a patient’s consciousness level. For instance, a score of 13-15 is classified as mild TBI; a score of 9-12 is classified as moderate TBI; a score of 8 and below is classified as severe TBI [24].
TBI and Neurodegenerative diseases
Neurodegenerative diseases are characterized by the loss of functional neurons in a debilitating and progressive manner, which can affect memory and physical capabilities. A history of TBI has been linked to induce long-term neurodegenerative diseases [5-7]. After TBI, diffuse axonal injury (DAI) is one of the most common neuropathological consequences; DAI results in vulnerable white matter axons [25-27]. Interestingly, in vitro and in vivo studies have found that myelinated axons are more tolerant to mechanical stress compared to unmyelinated axons [28-30]. The cascade of events seen in TBI pathophysiology is similar to the progression of neurodegeneration. Following trauma, inflammation intensifies; neuronal swelling occurs; and cytoskeletal failure and disconnection ultimately lead to Wallerian degeneration [31,32]. Wallerian degeneration is the innate immune response to traumatic nerve injury [33]. Neuron and axonal degeneration can have a role in the development of these diseases in both the acute and chronic phases following injury [34-36]. However, the risk of developing a neurodegenerative disease is partially dependent on the severity of the TBI. For instance, mild TBI without loss of consciousness does not necessarily correlate to enhanced risk of Parkinson’s (PD), Alzheimer’s (AD) or Huntington’s disease (HD) [37,38]. However, some TBI cases can lead to an earlier onset age of PD, AD, and HD [39-41]. The cause of PD symptoms is due to the loss of dopamine (DA) neurons, and thus DA neurotransmitters. While there are existing drugs that provide symptomatic relief to patients, there are no current treatments that attenuate disease progression. The overlap in neuropathology of TBI and neurodegenerative diseases suggest potential similar mechanisms. Thus, the trophic and immunomodulatory properties exhibited by MSCs may be applied to treat both TBI and neurodegenerative diseases.
MSCs and their Properties
Vocabulary
Stem cells are undifferentiated cells capable of self-renewal and proliferation that produce progeny cells which can then differentiate into various cell types. Stem cells are immature progenitor cells that divide via asymmetric mitosis, giving them self-renewal and differentiation capabilities. Asymmetric mitosis yields two daughter cells; one daughter cell is identical to the stem cell, while the second daughter cell is able to differentiate into a more mature cell [42]. There are several types of stem cells, but this review primarily focuses on MSCs. The International Society for Cellular Therapy has proposed criteria for the scientific community to accept to standardize the identification of MSCs [43]. MSCs are classified as heterogeneous multipotent stromal cells, meaning they are derived from embryonic cells that can differentiate into cells of the ectoderm, mesoderm and endoderm [44]. Ectodermal tissue includes neurons; mesodermal tissue includes adipocytes, osteocytes, and chondrocytes; endodermal tissue includes endothelial cells (EC) [45]. As the definition of MSCs states, MSCs are distinguished by particular surface markers. MSCs must be positive for CD105, CD73, and CD90, and negative for CD34, CD45, CD14, CD11b, CD7α, CD19, and HLA-DR surface molecules [46-48].
While MSCs are named stem cells, there has been an ongoing debate as to whether they actually qualify as stem cells; the accuracy of this title depends on the context of which they are being studied [49]. In vitro, MSCs have demonstrated their ability to proliferate, regenerate, and give rise to different progeny of ectodermal, mesodermal, and endodermal tissue descent. In vivo studies have also shown successful survival and differentiation into neurons and astrocytes, leading to improved motor function [50]. However, there are ongoing studies determining whether the phenotype of these stem cells may vary between in vitro and in vivo environments due to changing chemical and physical conditions [51]. This discussion is noteworthy to ensure research findings are not taken out of context. Yet, the science community has generally accepted MSCs as stem cells [51].
MSC sources
MSCs were originally isolated from bone marrow [52] but are also fetal and adult tissue of adipose tissue [13], placenta [53], lung [54], umbilical cord [55], synovial membrane [56], dental pulp [57], and many others. The properties of MSCs may vary depending on the source they are obtained from, cell culture conditions, and isolation procedures. In fact, variation has even been found in MSCs obtained from the same patient between isolations [42,58]. Some studies found that autologous MSCs differ from those obtained from healthy patients and can ultimately affect the efficacy of treatment [59]. Due to this, it is important to understand how these differences can affect MSC behavior when applied to clinical trials. Several studies aim to understand the properties of MSCs derived from bone marrow (BM-MSC), umbilical cord (UC-MSC), and adipose tissue (AT-MSC).
UC-MSCs generally have the highest proliferation rate regardless of the cell culture condition used [60,61]. In comparison, AT-MSCs have a moderate proliferation rate and BM-MSCs have the lowest proliferation rate [62-64]. However, BM-MSCs are most sufficient in maintaining their abundant secretion of trophic and proangiogenic factors independent of cell culture condition; AT-MSCs are the only producers of collagen I, II, and III; and UC-MSCs have the highest secretion of cytokines which attract inflammatory cells [62-65]. The production of collagen by AT-MSCs enhance the retention, survival, and brain metabolism of cells in rat TBI models [66]. UC-MSCs also reduced expression on inflammatory cytokines including IL-1α, IL-6, and IL-8 [61]. Differentiated cells can also influence levels of secreted cytokines, growth factors, proangiogenic factors, and proteins. For instance, without any stimulation, BM-MSCs already express neural proteins before differentiation even occurs.In particular, immature neuronal proteins such as Nestin and Tuj-1 were innately expressed; after exposure to a neuronal induction medium, mature neuronal proteins such as TH, MAP-2, and GFAP were significantly more expressed. This mature neuronal protein expression supports the differentiation capability of BM-MSCs into neurons and glial cells [67]. In addition, co-culturing BM-MSCs with astrocytes or Schwann cells further encourages differentiation into neurons and glial cells [68]. The trophic factors secreted by UC-MSCs differentiate microglia and macrophages to remodel the brain thus leading to significant improvement in neurological functioning [69]. UC-MSCs are also promising candidates for stem cell therapy as they are easily obtained, have a lower risk of rejection after transplantation, and no ethical controversy [70]. AT-MSCs are also easily isolated MSC derivatives and are capable of differentiating into neurons, endothelial-derived cells, and Schwann cells [68]. The neuroprotective properties of AT-MSCs are due to the release of growth factors, such as brain-derived neurotrophic factor (BDNF) and glial cell line-derived neurotrophic factor (GDNF). These subtle differences between MSC derived sources are critical to be aware of as they influence the final properties and efficacy when administered to patients.
Administration of MSCs to site of injury
The route of administration must be taken into consideration as it determines how many MSCs successful engraft to the site of injury. Three major methods of MSC delivery include intracranial [71], intraarterial (i.a.) [72] and intravenous (i.v.) infusion [73]. Other methods that have been used include intranasal [74-76], intrathecal [77], and intracisternal [78]. Intracranial injection provides a direct route for MSCs and shows the advantage of reducing the number of stem cells used but is also the most invasive. Knowing that the TBI and neurodegenerative diseases may not be localized, it could be deduced that systemic administration would have beneficial results. Both i.a. and i.v. administration have shown functional neural recovery in animal models [79-81], but the optimal administrational route has yet to be agreed on. Each method comes with advantages and disadvantages, for example, i.v. delivery can be used in broad distributions, large volumes, and is minimally invasive [82]; however, it is contradictory whether or not this method allows enough MSCs to reach the site of injury as most cells accumulate in the lungs, spleen, and liver [73,83-85]. On the contrary, the entrapped MSCs can then release micro vesicles and immunomodulatory factors that influence the healing process and state of the patient [83]. The i.a. method seems to direct the majority of injected MSCs to the brain, especially when injected through the carotid arteries [84,86]. However, there have been highly variable results using the i.a. route since precapillary entrapment can occur during i.a. infusion as well, but to a lesser degree than i.v. administration. Additionally, the i.a. route increased the risk of cerebral lesions after a stroke, which might limit the role of i.a. administration of MSCs [87]. Overall, the best route of administration has not been established as each method has advantages and disadvantages. Yet, the method of administration should be well-selected to treat TBI and neurodegeneration. While the delivery method is critical to consider, the survival of transplanted MSCs after transplantation is also crucial. Novel methods and mechanisms have been suggested to precondition stem cells in vitro in hopes of increasing the retention rates in vivo. Poor MSC survival in vivo has been linked to anoikis (type of programmed cell death), potential immune rejection, and oxidative damage mediating apoptosis [85].
The dose quantity is critical to consider in the administration process as well. One study showed that an increased number of MSCs administered does not show increased therapeutic effect in the brain injury animal model. Another study used autologous MSCs in hopes of increasing engraftment success after transplantation. A does of 107-109 cells were directly administered to the site of injury followed by a dose of 108-1010cells via i.v. infusion. There were no major adverse effects with exception of one patient who experienced two epilepsy episodes in the first two months [88]. The window of efficacy must be considered as well. One study done by Tian and others implanted MSCs via lumbar puncture into 97 patients, with 24 patients being in a permanent vegetative state [89]. The time of MSC administration varied between patients, but the overall finding was that the sooner MSCs are implanted to the time of injury, the more effective the treatment responses are likely to be.
Homing potentials
While the underlying mechanisms are unclear, MSCs contain a migratory capacity, allowing them to home to sites of injury [47, 90]; this property is crucial especially when MSCs are administered systemically. In other words, MSCs have the ability to recognize injured areas in otherwise healthy tissue. This homing potential of MSCs is influenced by chemokines, growth factors, and their ability to adhere to injured endothelium via vascular cell adhesion molecules [91]. Since MSCs may express a variety of chemokine receptors, the homing affinity could depend on the type of tissue [92]. One of the major pathways involved in MSC migration is the METR/HIF-1/CXCR4 pathway [93]. This pathway proceeds to decrease the number of peripheral leukocytes infiltrating the BBB; upregulates anti-inflammatory cytokines and downregulates proinflammatory cytokines; reduces the proinflammatory cytokine cascade; and ultimately slows and prevents the loss of neuronal cells. The chemo attractants involved in the enhancement of MSC homing to the brain are monocyte chemoattractant protein-1 (MCP-1) and stromal cell-derived factor-1 (SDF-1). Interestingly, the expression of these chemo attractants is dependent upon region and time. For instance, a study conducted by Lee and colleagues found that MCP-1 and SDF-1 directed i.v. infused MSCs to the cortex one day after injury or to the striatum in subsequent days [94].
Ability to cross blood-brain barrier
The blood-brain barrier (BBB) is a dynamic system critical to the central nervous system that separates peripheral blood from neural tissue. These blood vessels strictly regulate the movement of ions and molecules between the blood and brain. ECs in the BBB are predominantly responsible for its functions and interactions [95]. Thus, understanding the normal function and structure of this barrier is important when studying how it functions under variable conditions, such as disease or inflammation. MSCs exhibit leukocyte-like homing capabilities that enable them to interact with and cross the BBB. The BBB is naturally held together by tight junctions; it has been suggested that MSCs, similar to leukocytes, alter the properties of the tight junctions allowing them to infiltrate the brain [96]. There are many mechanisms involved in ensuring this crossing is successful [97]. MSCs utilize adhesion molecules (VCAM-1/VLA-4 and β1 integrin) to exit the bloodstream and adhere to the endothelium. MSCs then integrate into host tissue via the plasma podia [97]. Once in the brain, several studies support that MSCs already possess the ability to cross the BBB via paracellular pathways [96-99]. For instance, AT-MSCs administered intravenously were successful in crossing the BBB in mice with AD [100,101]. Successful MSC engraftment also displayed positive results in animal models with HD [102,103] and PD [104-106]. MSCs were observed to actively cross the BBB, but the alteration to the vasculature and inflammation post-injury may allow the passive movement and accumulation in the brain via entrapment [107]. TBI and neurodegenerative diseases break the impermeability of the BBB, allowing immune cells to enter the site of injury and trigger the release of inflammatory mediators [108,109]. This ability to cross the BBB is appealing for the treatment of TBI and neurodegenerative diseases.
MSC Differentiation
The multipotent differentiation capabilities of MSCs make this cell therapy promising for regenerative treatment. Differentiation into neuroectoderm is of particular interest here. During development, the neuroectoderm lies immediately above the notochord and gives rise to the entire nervous system; this includes the neural precursor cells, neural tube, brain, spinal cord, neurons, glial cells, and neural crest cells [110]. It has been confirmed that MSCs injected into the CNS migrate throughout the brain and adopt morphological and phenotypic characteristics of neurons and astrocytes (sub-type of glial cells) [111-114]. The numerous methods to promote neural cell differentiation is not well distinguished as they are inconsistent. However, it is suggested that expression of certain agents, neuronal proteins, secretion of growth factors, and environmental conditions play a role in neuronal differentiation of MSCs [110].
While MSC derivatives have an innate ability to differentiate, agents are generally used to induce neural differentiation in vitro. Such agents include compounds that increase intracellular cyclic AMP levels, retinoic acid, growth factors, antioxidants, a demethylating agent, and noggin (a physiological neural inducer); these agents collectively induce MSCs to adopt neuron-like morphologies and phenotypes as well as express several neuronal proteins [115]. Neuronal proteins expressed included nestin, glial fibrillary acidic protein (GFAP), neurofilament heavy chain (NF-H), and B-III tubulin [116,117]. Other studies have proposed methods to direct MSCs to differentiate into specific neuronal subtypes via expression of tyrosine hydroxylase [118-120], glutamate receptors [121], Schwann cell markers [122], glutamate transporters [123], inward rectifying potassium channels [124], synaptic vesicle proteins [125,126], and neurotrophic receptors [127]. Environmental conditions have also been suggested to play a part in influencing the differentiation of MSCs into neuroectoderm. For instance, MSC differentiation may require a toxic environment [128,129]. A toxic environment could include exposure to detergents, high pH, and molarity sodium chloride induced a neuron-like phenotype in MSCs [130].
One concern over the fact that so many agents can influence differentiation of MSCs is that cell fate decisions in development are generally controlled by one master regulatory gene [131]. Additionally, many of these processes are reversible. Thus, additional studies should be done to better understand if neural protein induction of MSCs alone constitutes functional differentiation and commitment into neuroectoderm in vivo, or whether they are just temporary behaviors in vitro.
Immunomodulatory Properties
Upon impact of injury or mechanism of neurodegeneration, the body activates its innate inflammatory response. Due to the homing potentials of MSCs, they are able to migrate to the site of injury and utilize their immunomodulatory properties to decrease inflammation. This reaction is crucial as excessive inflammation in the brain causes drastic degeneration of the central nervous system [132,133]. Furthermore, these kinds of injury activate microglia and astrocytes, which are primary sources of inflammatory cytokines, such as IL-1a, IL-1b, IL-6, TNF-a, and TNF-b [109,134]. If this cascade is not interrupted, the BBB is likely to be damaged, and be exacerbated as lymphocytes and monocytes continue to migrate to the site of injury [135,136]. Thus, the main immunological cells involved include microglia, astrocytes, lymphocytes, and macrophages [137,138]. MSCs modulate inflammation by upregulating anti-inflammatory signaling and deregulating pro-inflammatory signaling. MSCs also use direct cell-to-cell interactions and paracrine factor secretions to interfere with different pathways of the immune response [139-142]. For instance, MSCs have shown significant immunomodulatory effects such as inhibiting the proliferation of T cells, B cells, and natural killer cells [143-146]. Inflammation can also be modulated by MSC inhibition of interleukin six (IL-6) and IL1-b, pro-inflammatory signals, and enhancing IL-10, an anti-inflammatory signal [68, 147]. BM-MSCs exhibit anti-inflammatory effects by promoting the differentiation of lymphocytes. This modulatory behavior slows the inflammatory response cascade and as a result halts the progression of secondary brain injuries after impact. Therefore, MSCs may be a very promising treatment option to combat these detrimental events as they promote neurogenesis and endogenous repair.
Neurogenesis and Tissue Regeneration
Previously, it was thought that neurons were unable to regenerate, but stem cell research has revealed new potentials in the past two decades. Neuron development was generally understood to only occur during specific developmental periods. However, other studies have shown otherwise. Successful neurogenesis has been reported in the dentate gyrus of the hippocampus and the subventricular zone of the lateral ventricle during the postnatal and adult stages [148-150]. In order for MSCs to carry out neurogenesis and their tissue repair function, inflammation must be regulated as they secrete growth factors to maintain homeostasis. The trophic secretions of MSCs act as signaling molecules that induce functional tissue recovery by inhibiting apoptosis [151,152]; promoting neurogenesis [153-155], angiogenesis [156-158], and synaptogenesis [153-155]; and supporting the survival and proliferation of endogenous cells [149,159-161]. The significance of cytokine and adhesion molecule production by MSCs is well established to regulate hematopoiesis. Specifically, VEGF, BDNF, GDNF, and NGF, FGF-2, EGF, (NT-3) are leading factors in repairing lesioned areas [162-164]. GDNF promotes growth and nourishment to neurons lost in PD, particularly dopamine neurons. These chemokines may act indirectly by activating nearby astrocytes, which then promote neurogenesis [165]. Further studies revealed that MSCs encode proteins that regulate angiogenesis, wound repair, immunity, defense, and neural activities [166]. Interestingly, many of these proteins are expressed by subpopulations of cells; this complex composition explains the broad therapeutic applications of MSCs. As explained previously, MSCs are found in almost all adult human tissue; however, bone marrow is one of the most promising sources. One explanation for this is because bone and marrow are innervated by nervous tissue which is why BM-MSCs express several regulatory neuronal proteins including neurotrophins, neurite-inducing factors, and neuropeptides. These factors are understood to assist in guiding innervation to repair injured tissue as well as maintaining existing healthy tissue [167-169]. This behavior validates this approach for therapeutic treatment of TBI and neurodegenerative diseases.
Challenges and Future Prospects
While there are many promising qualities of MSCs in the treatment of TBIs and neurodegenerative diseases, there are many challenges that may affect actually applying this science in a clinical setting. For instance, more information is needed to understand how MSCs target specific tissues [170]; their potential correlation with tumors [171-174]; and the window of efficacy. The trophic factors of MSCs are capable of tissue regeneration, but the precise mechanisms and pathways need to be further understood. For instance, the secretory factors of MSCs are clearly beneficial in regenerative healing, but it should be distinguished whether these secretory factors can be used independently or if actual MSCs are necessary for regenerative treatment. With respect to concerns over the correlation to tumors, they may be prevented by use of MSC-derived exosomes [175]. With respect to the window of efficacy, MSC treatment is generally more effective when implemented close to the date of injury [176]. Yet, the challenge of understanding the effect of time on efficacy still needs to be overcome in patients receiving this treatment past the ideal window of care. In addition, there is a lack of standard parameters to measure the effectiveness of this therapy between MSC harvest location, TBI severity, and quantity of MSCs. Controls are also difficult to employ, placebo effects are abundant, and the cost of trials are great.
While the barriers mentioned above are significant, there is hope in the fact of knowing that similar problems have been encountered in previous novel treatments and have since been overcome. This objective may be accomplished by the combined efforts of hematologists and researchers around the world by creating a comprehensive website that presents all collected data of MSCs administered to patients [140]. Another aspect to consider is the necessity for a standardized cell injection strategy as repeated injections of MSCs have shown beneficial outcomes by providing continuous stimulation for repair [177-182]. There are two aspects that are the most striking in MSC treatment applications. First, few if any adverse effects have been well-documented in patients who received this treatment. Second, while many underlying mechanisms of this treatment are unclear, MSCs have resulted in drastic improvements in large scale animal models and some patients. Ultimately, the use of MSCs for the treatment of TBI and neurodegenerative disease is quite promising.
Conclusion
The use of MSCs for the treatment of TBI and neurodegenerative diseases has attracted substantial interest by the science community in the last two decades. While homing and differentiation capabilities of MSCs were the original attraction for therapeutic treatments, their release of paracrine molecules seem most promising due to the secretion of growth factors and anti-inflammatory effects. Both in vitro and in vivo experiments support trophic factors secreted by MSCs to modulate inflammation and create a more favorable environment for successful neurogenesis and tissue repair for TBI and neurodegenerative disease applications. More phase II/phase III trials are urgently needed to combat the challenges of this treatment and they should follow the guidelines proposed by the International Society for Stem Cell Research. Ultimately, the wide applications of MSCs provide hope to finding an effective and efficient therapeutic treatment option for TBIs and neurodegenerative diseases.
Acknowledgements
I would like to thank Dr. Gallicchio for his encouragement, constructive feedback, and guidance throughout this project. I would also like to dedicate this research review to my cousin, Brandi Pals.
References
- Vella MA, Crandall ML, Patel MB. (2017)Acute management of traumatic brain injury. SurgClin N AM. 97(5):1015-30.
- Coronado VG, McGuire LC, Faul M, Sugerman DE, Pearson WS. (2012) Traumatic brain injury epidemiology and public health issues. Brain injury medicine: Principles and practice. 84.
- Curry P, Viernes D, Sharma D. (2011) Perioperative management of traumatic brain injury. Int J CritIllnInj Sci. 1(1):27-35.
- Vardi G, Merrick J. (2008) Neurological disorders: Public health challenges. JPPID. 5(1):75.
- Plassman BL, Havlik RJ, Steffens DC, Helms MJ, Newman TN, et al. (2000) Documented head injury in early adulthood and risk of alzheimer's disease and other dementias. Neurol. 55(8):1158-66.
- Gardner RC, Yaffe K. (2014) Traumatic brain injury may increase risk of young onset dementia. Ann Neurol. 75(3):339.
- Gardner RC, Burke JF, Nettiksimmons J, Kaup A, Barnes DE, Yaffe K. (2014) Dementia risk after brain versus non-brain trauma: The role of age and severity. JAMA Neurol. 71(12):1490-7.
- Pierce AA, Xu AW. (2010) De novo neurogenesis in adult hypothalamus as a compensatory mechanism to regulate energy balance. J Neurosci. 30(2):723-30.
- Louveau A, Herz J, Alme MN, Salvador AF, Dong MQ, et al. (2018) CNS lymphatic drainage and neuroinflammation are regulated by meningeal lymphatic vasculature. Nature neurosci. (10):1380-91.
- Teixeira FG, Carvalho MM, Panchalingam KM, Rodrigues AJ, Mendes-Pinheiro B, et al. (2017) Impact of the secretome of human mesenchymal stem cells on brain structure and animal behavior in a rat model of parkinson's disease. SCTM. 6(2):634-46.
- Silva Meirelles L. (2006)Mesenchymal stem cells reside in virtually all post-natal organs and tissues. J Cell Sci. 19(11):2204-13.
- Urrutia DN, Caviedes P, Mardones R, Minguell JJ, Vega-Letter AM, et al. (2019) Comparative study of the neural differentiation capacity of mesenchymal stromal cells from different tissue sources: An approach for their use in neural regeneration therapies. PloS One. 14(3):e0213032.
- Zuk PA, Zhu M, Mizuno H, Huang J, Futrell JW, et al. (2001)Multilineage cells from human adipose tissue: Implications for cell-based therapies. Tissue Engineer. 7(2):211-28.
- Erices A, Conget P, Minguell JJ. (2000)Mesenchymal progenitor cells in human umbilical cord blood. Br J Haematol. 109(1):235-42.
- Liu L, Eckert MA, Riazifar H, Kang DK, Agalliu D, et al. (2013)From blood to the brain: Can systemically transplanted mesenchymal stem cells cross the blood-brain barrier? Stem Cells Int. 2013:1-7.
- Zhang Y, Chopp M, Meng Y, Katakowski M, Xin H, et al. (2015) Effect of exosomes derived from multipluripotentmesenchymal stromal cells on functional recovery and neurovascular plasticity in rats after traumatic brain injury. J Neurosurg. 122(4):856-67.
- Wang Z, Wang Y, Wang Z, Gutkind JS, Wang Z, et al. (2015) Engineered mesenchymal stem cells with enhanced tropism and paracrine secretion of cytokines and growth factors to treat traumatic brain injury. Stem Cells (Dayton, Ohio). 33(2):456-67.
- Algattas H, Huang JH. (2013) Traumatic brain injury pathophysiology and treatments: Early, intermediate, and late phases post-injury. Int J Mol Sci. 15(1):309-41.
- Johnson VE, Stewart W, Smith DH. (2013) Axonal pathology in traumatic brain injury. Experiment Neurol. 246:35-43.
- Xiong Y, Gu Q, Peterson Pl, Muizelaar JP, Lee CP. (1997) Mitochondrial dysfunction and calcium perturbation induced by traumatic brain injury. J Neurotrauma. 14(1):23-34.
- Maas AI, Stocchetti N, Bullock R. (2008) Moderate and severe traumatic brain injury in adults. Lancet Neurol. 7(8):728.
- Avellino AM, Hart D, Dailey AT, MacKinnon M, Ellegala D, et al. (1995) Differential macrophage responses in the peripheral and central nervous system during wallerian degeneration of axons. Experiment Neurol. 136(2):183-98.
- Rolls A, Shechter R, Schwartz M. (2009) The bright side of the glial scar in CNS repair. Nature Rev Neurosci. 10(3):235-41.
- Langlois JA, Rutland-Brown W, Wald MM. (2006)The epidemiology and impact of traumatic brain injury: a brief overview. J Head Trauma Rehabil. 21(5):375-8.
- Adams JH, Doyle D, Ford I, Gennarelli TA, Graham DI, et al. (1989) Diffuse axonal injury in head injury: Definition, diagnosis and grading. Histopathol. 15(1):49-59.
- Adams JH, Graham DI, Murray LS, Scott G. (1982)Diffuse axonal injury due to nonmissile head injury in humans: An analysis of 45 cases. Ann Neurol. 12(6):557-63.
- Povlishock JT, Christman CW. (1995)The pathobiology of traumatically induced axonal injury in animals and humans: A review of current thoughts. J Neurotrauma. 12(4):555-64.
- Reeves TM, Phillips LL, Lee NN, Povlishock JT. (2007) Preferential neuroprotective effect of tacrolimus (FK506) on unmyelinated axons following traumatic brain injury. Brain Res. 1154:225-36.
- Reeves TM, Phillips LL, Povlishock JT. (2005)Myelinated and unmyelinated axons of the corpus callosum differ in vulnerability and functional recovery following traumatic brain injury. Experiment Neurol. 196(1):126-37.
- Staal JA, Vickers JC. (2011) Selective vulnerability of non-myelinated axons to stretch injury in an in vitro co-culture system. J Neurotrauma. 28(5):841-7.
- Povlishock JT, Becker DP. (1985) Fate of reactive axonal swellings induced by head injury. Lab Invest. 52(5):540-52.
- Povlishock JT, Katz DI. (2005) Update of neuropathology and neurological recovery after traumatic brain injury. J Head Trauma Rehabil. 20(1):76-94.
- Rotshenker S. (2011)Wallerian degeneration: The innate-immune response to traumatic nerve injury. J Neuroinflam. 8(1):109.
- Smith DH, Stewart W. (2018) Traumatic brain injury: A platform for studies in aβ processing. Brain Pathology (Zurich, Switzerland) 28(4):463-5.
- Chen X, Johnson VE, Uryu K, Trojanowski JQ, Smith DH. (2009) A lack of amyloid β plaques despite persistent accumulation of amyloid β in axons of long-term survivors of traumatic brain injury. Br Pathol (Zurich, Switzerland) 19(2):214-23.
- Chen XH, Siman R, Iwata A, Meaney DF, Trojanowski JQ, et al. (2004) Long-term accumulation of amyloid-, -secretase, presenilin-1, and caspase-3 in damaged axons following brain trauma materials and methods. Am J Pathol. 165(2):357-71.
- Godbolt AK, Cancelliere C, Hincapie CA, Marras C, Boyle E, et al. (2014) Systematic review of the risk of dementia and chronic cognitive impairment after mild traumatic brain injury: Results of the international collaboration on mild traumatic brain injury prognosis. Arch Phys Med Rehabil. 95(3).
- Bower JH, Maraganore DM, Peterson BJ, McDonnell SK, Ahlskog JE, et al. Head trauma preceding PD: A case-control study. Neurol. 60(10):1610-5.
- Sullivan P, Petitti D, Barbaccia J. (1987) Head trauma and age of onset of dementia of the alzheimer type. JAMA: JAMAAP. 257(17):2289-90.
- Nemetz PN, Leibson C, Naessens JM, Beard M, Kokmen E, et al. (1999) Traumatic brain injury and time to onset of alzheimer's disease: A population-based study. Am J Epidemiol. 149(1):32-40.
- Maher NE, Golbe LI, Lazzarini AM, Mark MH, Currie LJ, et al. (2002) Epidemiologic study of 203 sibling pairs with parkinson's disease: The GenePD study. Neurol. 58(1):1136.
- Elahi KC, Klein G, Avci-Adali M, Sievert KD, MacNeil S, et al. (2016) Human mesenchymal stromal cells from different sources diverge in their expression of cell surface proteins and display distinct differentiation patterns. Stem Cells Int. 2016:1-9.
- Dominici M, Le Blanc K, Mueller I, Slaper-Cortenbach I, Marini FC, et al. (2006) Minimal criteria for defining multipotentmesenchymal stromal cells. the international society for cellular therapy position statement. Cytotherapy (Oxford, England). 8(4):315-7.
- Phinney DG, Prockop DJ. (2007) Concise review: Mesenchymal stem/multipotent stromal cells: The state of transdifferentiation and modes of tissue repair-current views. Stem Cells (Dayton, Ohio) 25(11):2896-902.
- Caplan AI. (2005) Review: Mesenchymal stem cells: Cell-Based reconstructive therapy in orthopedics. Tissue Engin. 11(7-8):1198-211.
- Ding DC, Shyu WC, Lin SZ. (2011)Mesenchymal stem cells. Cell transplant. 20(1):5-14.
- Devine SM, Bartholomew AM, Mahmud N, Nelson M, PatilS, et al. (2011)Mesenchymal stem cells are capable of homing to the bone marrow of non-human primates following systemic infusion. Experiment Hematol. 02;29(2):244.
- Dominici M, Le Blanc K, Mueller I, Slaper-Cortenbach I, Marini FC, et al. (2006) Minimal criteria for defining multipotentmesenchymal stromal cells. the international society for cellular therapy position statement. Cytotherapy (Oxford, England). 8(4):315-7.
- Kolf CM, Cho E, Tuan RS. (2007) Biology of adult mesenchymal stem cells: Regulation of niche, self-renewal and differentiation. BioMed Central Ltd. 204(9):1-10.
- Mahmood A, Lu D, Li Y, Chen JL, Chopp M. (2010) Intracranial bone marrow transplantation after traumatic brain injury improving functional outcome in adult rats. J Neurosurg. 94(4):589-95.
- Augello A, Kurth TB, De Bari C. (2010)Mesenchymal stem cells: A perspective from in vitro cultures to in vivo migration and niches. Eur Cells Mater. 20:121-33.
- (52)Friedenstein AJ, Chailakhjan RK, Lalykina KS. (1970)The development of fibroblast colonies in monolayer cultures of guinea-pig bone marrow and spleen cells. Cell Prolifer. 3(4):393-403.
- Miao Z, Jin J, Chen L, Zhu J, Huang W, et al. (2006) Isolation of mesenchymal stem cells from human placenta: Comparison with human bone marrow mesenchymal stem cells. Cell Biol Int. 30(9):681-7.
- Griffiths MJ, Bonnet D, Janes SM. (2005) Stem cells of the alveolar epithelium. The Lancet. 366(9481):249.
- Wang H, Hung S, Peng S, Huang C, Wei H, et al. (2004)Mesenchymal stem cells in the wharton's jelly of the human umbilical cord. Stem Cells (Dayton, Ohio). 22(7):1330-7.
- De Bari C, Dell'Accio F, Tylzanowski P, Luyten FP. (2001)Multipotentmesenchymal stem cells from adult human synovial membrane. Arthritis and Rheumatism. 44(8):1928-42.
- Gronthos S, Mankani M, Brahim J, Robey PG, Shi S. (2000)Postnatal human dental pulp stem cells (DPSCs) in vitro and in vivo. Proceedings of the National Academy of Sciences-PNAS. 97(25):13625-30.
- Fuchs, Tumbar, Guasch. (2004) Review socializing with the neighbors: Stem cells and their niche fied by experiments in which the fate of ESCs is monitored following their subcutaneous injection into nude mice. ESCs isolated from a blastocyst-stage mouse em. Cell. 116(6):769-78.
- Koh S, Baik W, Noh MY, Cho GW, Kim HY, et al. (2012) The functional deficiency of bone marrow mesenchymal stromal cells in ALS patients is proportional to disease progression rate. Experiment Neurol. 233(1):472-80.
- Fazzina R, Iudicone P, Fioravanti D, Bonanno G, Totta P, et al. (2016) Potency testing of mesenchymal stromal cell growth expanded in human platelet lysate from different human tissues. Stem Cell Res Ther. 7(1):122.
- Jin H, Bae Y, Kim M, Kwon S, Jeon H, et al. (2013) Comparative analysis of human mesenchymal stem cells from bone marrow, adipose tissue, and umbilical cord blood as sources of cell therapy. Int J Mol Sci. 14(9):17986-8001.
- Amable PR, Teixeira MVT, Carias RBV, Granjeiro JM, Borojevic R. (2014)Mesenchymal stromal cell proliferation, gene expression and protein production in human platelet-rich plasma-supplemented media. PloS One. 9(8):e104662.
- RominaAmable P, Vinicius M, Teixeira T, Bizon R, Carias V, et al. Gene expression and protein secretion during human mesenchymal cell differentiation into adipogenic cells. BMC cell biol. 5(1):1.
- Amable PR, Teixeira MVT, Carias RBV, Granjeiro JM, Borojevic R. (2014) Protein synthesis and secretion in human mesenchymal cells derived from bone marrow, adipose tissue and wharton’s jelly. Stem Cell Res Ther. 5(2):1-3.
- Balasubramanian S, Thej C, Venugopal P, Priya N, Zakaria Z, et al. (2013) Higher propensity of wharton's jelly derived mesenchymal stromal cells towards neuronal lineage in comparison to those derived from adipose and bone marrow. Cell Biol Int. 37(5):507-15.
- Guan J, Zhu Z, Zhao RC, Xiao Z, Wu C, et al. (2013) Transplantation of human mesenchymal stem cells loaded on collagen scaffolds for the treatment of traumatic brain injury in rats. Biomat. 34(24):5937-46.
- Sekiya I, Larson BL, Smith JR, Pochampally R, Cui J, et al. (2002) Expansion of human adult stem cells from bone marrow stroma: Conditions that maximize the yields of early progenitors and evaluate their quality. Stem Cells (Dayton, Ohio). 20(6):530-41.
- Ghasemi, Ghasemi. (2018)Transdifferentiation of human adipose-derived mesenchymal stem cells into oligodendrocyte progenitor cells. Iran J Neurol. 17(1):24.
- Chen KH, Shao PL, Li YC, Chiang JY, Sung PH, ert al. (2020) Human umbilical cord-derived mesenchymal stem cell therapy effectively protected the brain architecture and neurological function in rat after acute traumatic brain injury. Cell Transplant. 29:963689720929313.
- Romanov YA. (2003) Searching for alternative sources of postnatal human mesenchymal stem cells: Candidate MSC-like cells from umbilical cord. Stem Cells (Dayton, Ohio). 21(1):105-10.
- Mahmood A, Lu D, Wang L, Chopp M. (2002)Intracerebral transplantation of marrow stromal cells cultured with neurotrophic factors promotes functional recovery in adult rats subjected to traumatic brain injury. J Neurotrauma. 19(12):1609-17.
- Lu D, Li Y, Wang L, Chen J, Mahmood A, et al. (2001)Intraarterial administration of marrow stromal cells in a rat model of traumatic brain injury. J Neurotrauma. 18(8):813-9.
- Lu D, Mahmood A, Wang L, Li Y, Lu M, et al. (2001) Adult bone marrow stromal cells administered intravenously to rats after traumatic brain injury migrate into brain and improve neurological outcome. Neuroreport. 12(3):559-63.
- Chau MJ, Deveau TC, Gu X, Kim YS, Xu Y, et al. (2018) Delayed and repeated intranasal delivery of bone marrow stromal cells increases regeneration and functional recovery after ischemic stroke in mice. BMC Neurosci. 19(1):20.
- Das M, Mayilsamy K, Tang X, Han JY, Foran E, et al. (2019) Pioglitazone treatment prior to transplantation improves the efficacy of human mesenchymal stem cells after traumatic brain injury in rats. Scientific Rep. 9(1):13646-13.
- Donega V, Nijboer CH, van Velthoven, Cindy TJ, Youssef SA, et al. (2015) Assessment of long-term safety and efficacy of intranasal mesenchymal stem cell treatment for neonatal brain injury in the mouse. Pediat Res. 78(5):520-6.
- Vaquero J, Zurita M, Bonilla C, Fernandez C, Rubio JJ, et al. (2016) Progressive increase in brain glucose metabolism after intrathecal administration of autologous mesenchymal stromal cells in patients with diffuse axonal injury. Cytother. 19(1):88-94.
- Shin DA, Kim J, Kim H, Yi S, Ha Y, et al. (2013) Comparison of functional and histological outcomes after intralesional, intracisternal, and intravenous transplantation of human bone marrow-derived mesenchymal stromal cells in a rat model of spinal cord injury. ActaNeurochir. 155(10):1943-50.
- Giraldi-Guimaraes A, Rezende-Lima M, Bruno FP, Mendez-Otero R. (2009) Treatment with bone marrow mononuclear cells induces functional recovery and decreases neurodegeneration after sensorimotor cortical ischemia in rats. Brain Res. 1266:108-20.
- Dos Santos AD, da Costa Reis J, Paredes BD, Moraes L, Giraldi-Guimaraes A, et al. (2009) Therapeutic window for treatment of cortical ischemia with bone marrow-derived cells in rats. Brain Res. 1306:149-58.
- Brenneman M, Sharma S, Harting M, Strong R, Cox CS, et al. (2010) Autologous bone marrow mononuclear cells enhance recovery after acute ischemic stroke in young and middle-aged rats. J Cereb Blood Flow Metab. 30(1):140-9.
- Mahmood A, Lu D, Wang L, Li Y, Lu M, Chopp M. (2001) Treatment of traumatic brain injury in female rats with intravenous administration of bone marrow stromal cells. Neurosurg. 49(5):1196-204.
- Agadi S, Shetty AK. (2015) Concise review: Prospects of bone marrow mononuclear cells and mesenchymal stem cells for treating status epilepticus and chronic epilepsy. Stem Cell. 33(7):2093.
- Silachev DN, Plotnikov EY, Babenko VA, Danilina TI, Zorov LD, et al. (2009) Intra-arterial administration of multipotentmesenchymal stromal cells promotes functional recovery of the brain after traumatic brain injury. Bull ExpBiol Med. 159(4):528.
- Sart S, Ma T, Li Y. (2014) Preconditioning stem cells for in vivo delivery. BioRes Open Access. 3(4):137-49.
- Walczak P, Zhang J, Gilad AA, Kedziorek DA, Ruiz-Cabello J, et al. (2008) Dual-modality monitoring of targeted intraarterial delivery of mesenchymal stem cells after transient ischemia. Stroke. 39(5):1569-74.
- Zhang Z, Guan L, Zhang K, Zhang Q, Dai L. (2008) A combined procedure to deliver autologous mesenchymal stromal cells to patients with traumatic brain injury. Cytotherapy (Oxford, England). 10(2):134-9.
- Tian C, Wang X, Wang X, Wang L, Wang X, et al. (2013)Autologous bone marrow mesenchymal stem cell therapy in the subacute stage of traumatic brain injury by lumbar puncture. Experiment Clinic Transplant. 11(2):176-81.
- Devine SM, Cobbs C, Jennings M, Bartholomew A, Hoffman R. (2003)Mesenchymal stem cells distribute to a wide range of tissues following systemic infusion into nonhuman primates. Blood. 101(8):2999-3001.
- Devine SM, Cobbs C, Jennings M, Bartholomew A, Hoffman R. (2003)Mesenchymal stem cells distribute to a wide range of tissues following systemic infusion into non-human primates. Blood. 101(8):2999-3001.
- Chute J. (2006) Stem cell homing. Current Opinion in Hematology. 13(6):399-406.
- Luttichau IV, Notohamiprodjo M, Wechselberger A, Peters C, Henger A, et al. (2005) Human adult CD34 − progenitor cells functionally express the chemokine receptors CCR1, CCR4, CCR7, CXCR5, and CCR10 but not CXCR4. Stem Cells Develop. 14(3):329-36.
- Bang OY, Gyeong J, Moon DH, Kim JH, Lee S, et al. (2017) The STARTING-2 trial investigators. Translat Stroke Res. 8:449.
- Lee SH, Jin KS, Bang OY, Kim BJ, Park SJ, et al. (2015) Differential migration of mesenchymal stem cells to ischemic regions after middle cerebral artery occlusion in rats. PloS One. 10(8):e0134920.
- Daneman R, Prat A. (2015) The Blood-Brain barrier. Cold Spring Harbor Perspectives in Biology. 7(1):a020412.
- Matsushita T, Kibayashi T, Katayama T, Yamashita Y, Suzuki S, et al. (2011)Mesenchymal stem cells transmigrate across brain microvascular endothelial cell monolayers through transiently formed inter-endothelial gaps. Neurosci Letters. 502(1):41-5.
- Steingen C, Brenig F, Baumgartner L, Schmidt J, Schmidt A, et al. (2008) Characterization of key mechanisms in transmigration and invasion of mesenchymal stem cells. J Mol Cell Cardiol. 44(6):1072-84.
- Conaty P, Sherman LS, Naaldijk Y, Ulrich H, Stolzing A, et al. (2018) Methods of mesenchymal stem cell homing to the Blood-Brain barrier. New York, NY: Springer New York.
- Schmidt A, Ladage D, Steingen C, Brixius K, Schinkothe T, et al. (2006)Mesenchymal stem cells transmigrate over the endothelial barrier. Eur J Cell Biol. 85(11):1179-88.
- Vargas-Restrepo F, Sabogal-Guaqueta AM, Cardona-Gómez GP. (2018)Quercetin ameliorates inflammation in CA1 hippocampal region in aged triple transgenic alzheimer's disease mice model. Biomedica. 38(suppl 1):69-76.
- Jeon D, Chu K, Lee S, Jung K, Kang K, et al. (2011)A cell‐free extract from human adipose stem cells protects mice against epilepsy. Epilepsia (Copenhagen). 52(9):1617-26.
- Freeman TB, Cicchetti F, Hauser RA, Deacon TW, Li XJ, et al. (2000) Transplanted fetal striatum in huntington's disease: Phenotypic development and lack of pathology. Proceedings of the National Academy of Sciences-PNAS. 97(25):13877-82.
- Bjorklund A LO. (2000) Cell replacement therapies for central nervous system disorders. Nature Neurosci. 6(3):537-44.
- Weimann JM, Charlton CA, Brazelton TR, Hackman RC, Blau HM. (2003) Contribution of transplanted bone marrow cells to purkinje neurons in human adult brains. Proceedings of the National Academy of Sciences-PNAS. 100(4):2088-93.
- Ringe J, Kaps C, Burmester G, Sittinger M. (2002) Stem cells for regenerative medicine: Advances in the engineering of tissues and organs. Die Naturwissenschaften. 89(8):338-51.
- Dezawa M, Kanno H, Hoshino M, Cho H, Matsumoto N, et al. (2004) Specific induction of neuronal cells from bone marrow stromal cells and application for autologous transplantation. J Clin Invest. 113(12):1701-10.
- Lee RH, Pulin AA, Seo MJ, Kota DJ, YlostaloJ,et al. (2009) Intravenous hMSCs improve myocardial infarction in mice because cells embolized in lung are activated to secrete the anti-inflammatory protein TSG-6. Cell Stem Cell. 5(1):54-63.
- Werner C, Engelhard K. (2007) Pathophysiology of traumatic brain injury. Br J Anaesth. 99(1):4-9.
- Ziebell JM, Morganti-Kossmann MC. (2010) Involvement of pro- and anti-inflammatory cytokines and chemokines in the pathophysiology of traumatic brain injury. Neurotherap. 7(1):22-30.
- Resources how to GETTING STARTED NCBI education NCBI help manual NCBI handbook.
- Kopen GC, Prockop DJ, Phinney DG. (1999) Marrow stromal cells migrate throughout forebrain and cerebellum, and they differentiate into astrocytes after injection into neonatal mouse brains. Proceedings of the National Academy of Sciences-PNAS. 96(19):10711-6.
- Chen J, Li Y, Wang L, Zhang Z, Lu D, et al. (2001) Therapeutic benefit of intravenous administration of bone marrow stromal cells after cerebral ischemia in rats. Stroke (1970). 32(4):1005-11.
- Zhao L, Duan W, Reyes M, Keene CD, Verfaillie CM, et al. (2002) Human bone marrow stem cells exhibit neural phenotypes and ameliorate neurological deficits after grafting into the ischemic brain of rats. Experiment Neurol. 174(1):11-20.
- Munoz-Elias G. (2004) Adult bone marrow stromal cells in the embryonic brain: Engraftment, migration, differentiation, and long-term survival. J Neurosci. 24(19):4585-95.
- Nakano K, Migita M, Mochizuki H, Shimada T. (2001) Differentiation of transplanted bone marrow cells in the adult mouse brain 1. Transplant. 71:1735.
- Krabbe C, Zimmer J, Meyer M. (2005)Neuraltransdifferentiation of mesenchymal stem cells-a critical review. APMIS: ActaPathologica, MicrobiologicaEtImmunologicaScandinavica. 113(11-12):831-44.
- Juan R Sanchez-Ramos. (2002)Neural cells derived from adult bone marrow and umbilical cord blood. J Neurosci Res. 69(6):880-93.
- Fu Y, Cheng Y, Lin MA, Cheng H, Chu P, et al. (2006) Conversion of human umbilical cord mesenchymal stem cells in wharton's jelly to dopaminergic neurons in vitro: Potential therapeutic application for parkinsonism. Stem Cells (Dayton, Ohio). 24(1):115-24.
- Tao H, Rao R, Ma DDF. (2005) Cytokine‐induced stable neuronal differentiation of human bone marrow mesenchymal stem cells in a serum/feeder cell‐free condition. Development, Growth & Differentiation. 47(6):423-33.
- Khademizadeh M, Messripour M, Ghasemi N, Beik FM, Attar AM. (2019) Differentiation of adult human mesenchymal stem cells into dopaminergic neurons. Res Pharm Sci. 14(3):209-15.
- Kondo T, Johnson SA, Yoder MC, Romand R, Hashino E. (2005) Sonic hedgehog and retinoic acid synergistically promote sensory fate specification from bone marrow-derived pluripotent stem cells. Proceedings of the National Academy of Sciences. 102(13):4789-94.
- Jenny Caddick, Paul J Kingham, Natalie J Gardiner, Mikael Wiberg, Giorgio Terenghi. (2006) Phenotypic and functional characteristics of mesenchymal stem cells differentiated along a schwann cell lineage. Glia. 54(8):840-9.
- De Hemptinne I, Vermeiren C, Maloteaux J, Hermans E. (2004) Induction of glial glutamate transporters in adult mesenchymal stem cells. J Neurochem. 91(1):155-66.
- Mareschi K, Novara M, Rustichelli D, Ferrero I, Guido D. (2006) Neural differentiation of human mesenchymal stem cells: Evidence for expression of neural markers and eag K+ channel types. Experiment Hematol. 34(11):1563-72.
- Cho KJ, Trzaska KA, Greco SJ, McArdle J, Wang FS, et al. (2005) Neurons derived from human mesenchymal stem cells show synaptic transmission and can be induced to produce the neurotransmitter substance P by Interleukin‐1α. Stem Cells (Dayton, Ohio). 23(3):383-91.
- Woodbury D, Reynolds K, Black IB. (2002) Adult bone marrow stromal stem cells express germline, ectodermal, endodermal, and mesodermal genes prior to neurogenesis. J Neurosci Res. 69(6):908-17.
- Shahbazi S, Alikarami K, Asadi TJ, Soleimani I. (2016) I IJ JM MC CM M rapid induction of neural differentiation in human umbilical cord matrix mesenchymal stem cells by cAMP-elevating agents.
- Croft AP, Przyborski SA. (2006) Formation of neurons by non-neural adult stem cells: Potential mechanism implicates an artifact of growth in culture. Stem Cells (Dayton, Ohio). 24(8):1841-51.
- Lu P, Blesch A, Tuszynski MH. (2004) Induction of bone marrow stromal cells to neurons: Differentiation, transdifferentiation, or artifact? J Neurosci Res. 77(2):174-91.
- Odom DT. (2004) Control of pancreas and liver gene expression by HNF transcription factors. Science (American Association for the Advancement of Science). 303(5662):1378-81.
- Loane DJ, Kumar A. (2016) Microglia in the TBI brain: The good, the bad, and the dysregulated. Experiment Neurol. 275(3):316-27.
- Simon DW, McGeachy MJ, Bayır H, Clark RSB, Loane DJ, et al. (2017)The far-reaching scope of neuroinflammation after traumatic brain injury. Nature Reviews. Neurol. 13(3):171-91.
- Wang CX, Shuaib A. (2002) Involvement of inflammatory cytokines in central nervous system injury. Progress in Neurobiol. 67(2):161-72.
- Obermeier B, Daneman R, Ransohoff RM. (2013) Development, maintenance and disruption of the blood-brain barrier. Nature Med. 19(12):1584-96.
- Simi A, Tsakiri N, Wang P, Rothwell NJ. (2007) Interleukin-1 and inflammatory neurodegeneration. Biochem Society Transact. 35(Pt 5):1122-6.
- Ma S, Xie N, Li W, Yuan B, Shi Y, et al. (2013)Immunobiology of mesenchymal stem cells. Cell Death and Differentiation. 21(2):216-25.
- Di Trapani M, Bassi G, Ricciardi M, Fontana E, Bifari F, et al. Comparative study of immune regulatory properties of stem cells derived from different tissues. Stem Cells and Development. 22(22):2990-3002.
- Prockop DJ, Youn Oh J. (2012)Mesenchymal stem/stromal cells (MSCs): Role as guardians of inflammation. MolTher. 20(1):14-20.
- Prockop DJ. (2017)The exciting prospects of new therapies with mesenchymal stromal cells. Cytotherapy (Oxford, England). 19(1):1-8.
- Connick P, Kolappan M, Crawley C, Webber DJ, Patani R, et al. (2012) Autologous mesenchymal stem cells for the treatment of secondary progressive multiple sclerosis: An open-label phase 2a proof-of-concept study. Lancet Neurol. 11(2):150.
- Wilson JG, Liu KD, Zhuo H, Caballero L, Mcmillan M, et al. Mesenchymal stem (stromal) cells for treatment of ARDS: A phase 1 clinical trial. Lancet Resp Med. 3(1):24.
- Beggs KJ, Lyubimov A, Borneman JN, Bartholomew A, Moseley A, et al. (2006) Immunologic consequences of multiple, high-dose administration of allogeneic mesenchymal stem cells to baboons. Cell Transplant. 15(8-9):711-21.
- Glennie S, Soeiro I, Dyson PJ, Lam EW, Dazzi F. (2005) Bone marrow mesenchymal stem cells induce division arrest anergy of activated T cells. Blood. 105(7):2821-7.
- Keyser KA, Beagles KE, Kiem H. (2017) Comparison of mesenchymal stem cells from different tissues to suppress T-cell activation. Cell Transplant. 16(5):555-62.
- Martinez HR, Molina-Lopez JF, Gonzalez-Garza MT, Moreno-Cuevas JE, Caro-Osorio E, et al. (2012) Stem cell transplantation in amyotrophic lateral sclerosis patients: Methodological approach, safety, and feasibility. Cell Transplant. 21(9):1899-907.
- Ma H, Lam PK, Tong CSW, Lo KKY, Wong GKC, et al. (2019) The neuroprotection of hypoxic adipose tissue-derived mesenchymal stem cells in experimental traumatic brain injury. Cell Transplant. 28(7):874-84.
- Danielyan L, Schafer R, von Ameln-Mayerhofer A, Buadze M, Geisler J, et al. (2009) Intranasal delivery of cells to the brain. Eur J Cell Biol. 88(6):315-24.
- Munoz JR, Stoutenger BR, Robinson AP, Spees JL, Prockop DJ. (2005) Human stem͞progenitor cells from bone marrow promote neurogenesis of endogenous neural stem cells in the hippocampus of mice. Proceedings of the National Academy of Sciences. 102(50):18171-6.
- Kassis I, Grigoriadis N, Gowda-Kurkalli B, Mizrachi-Kol R, Ben-Hur T, et al. (2008)Neuroprotection and immunomodulation with mesenchymal stem cells in chronic experimental autoimmune encephalomyelitis. Archives of Neurology (Chicago). 65(6):753-61.
- Inoue Y, Iriyama A, Ueno S, Takahashi H, Kondo M, et al. (2007)Subretinal transplantation of bone marrow mesenchymal stem cells delays retinal degeneration in the RCS rat model of retinal degeneration. Experiment Eye Res. 85(2):234-41.
- Hung S, Pochampally RR, Chen S, Hsu S, Prockop DJ. (2007)Angiogenic effects of human multipotent stromal cell conditioned medium activate the PI3K-akt pathway in hypoxic endothelial cells to inhibit apoptosis, increase survival, and stimulate angiogenesis. Stem Cells (Dayton, Ohio). 25(9):2363-70.
- Li Y, Chopp M. (2009) Marrow stromal cell transplantation in stroke and traumatic brain injury. Neurosci Letters. 456(3):120-3.
- Aertker BM, Bedi S, Cox CS. (2016) Strategies for CNS repair following TBI. Experiment Neurol. 275:411-26.
- Xiong Y, Mahmood A, Chopp M. (2010) Angiogenesis, neurogenesis and brain recovery of function following injury. Current Opinion in Investigational Drugs (London, England: 2000). 11(3):298-308.
- Shyu K, Wang B, Hung H, Chang C, Tzu-Bi D, Shih DT. Mesenchymal stem cells are superior to angiogenic growth factor genes for improving myocardial performance in the mouse model of acute myocardial infarction. J Biomed Sci. 13(1):47-58.
- Tang J, Xie Q, Pan G, Wang J, Wang M. (2006)Mesenchymal stem cells participate in angiogenesis and improve heart function in rat model of myocardial ischemia with reperfusion. Eur J Cardiothorac Surg. 30(2):353-61.
- Zwezdaryk KJ, Coffelt SB, Figueroa YG, Liu J, Phinney DG, et al. (2007) Erythropoietin, a hypoxia-regulated factor, elicits a pro-angiogenic program in human mesenchymal stem cells. Experiment Hematol. 35(4):640-52.
- Lee RH, Seo MJ, Reger RL, Spees JL, Pulin AA, et al. (2006)Multipotent stromal cells from human marrow home to and promote repair of pancreatic islets and renal glomeruli in diabetic NOD͞scid mice. 103(46):17438-43.
- Mahmood A, Lu D, Chopp M. (2004) Marrow stromal cell transplantation after traumatic brain injury promotes cellular proliferation within the brain. Neurosurg. 55(5):1185-93.
- Crigler L, Robey RC, Asawachaicharn A, Gaupp D, Phinney DG. (2006) Human mesenchymal stem cell subpopulations express a variety of neuro-regulatory molecules and promote neuronal cell survival and neuritogenesis. Experiment Neurol. 198(1):54-64.
- Zhang J, Li Y, Chen J, Yang M, Katakowski M, et al. (2004) Expression of insulin-like growth factor 1 and receptor in ischemic rats treated with human marrow stromal cells. Brain Res. 1030(1):19-27.
- Uccelli A, Benvenuto F, Laroni A, Giunti D. (2011)Neuroprotective features of mesenchymal stem cells. Best Practice & Research. Clinic Haematol. 24(1):59-64.
- Munoz JR, Stoutenger BR, Robinson AP, Spees JL, Prockop DJ. (2002) Human stem͞progenitor cells from bone marrow promote neurogenesis of endogenous neural stem cells in the hippocampus of mice. 102(50):18171-6.
- Song H, Stevens CF, Gage FH. (2002)Astroglia induce neurogenesis from adult neural stem cells. Nature (London). 417(6884):39-44.
- Tremain N, Korkko J, Ibberson D, Kopen GC, DiGirolamo C, et al. (2001)MicroSAGE analysis of 2,353 expressed genes in a single Cell‐Derived colony of undifferentiated human mesenchymal stem cells reveals mRNAs of multiple cell lineages. Stem Cells (Dayton, Ohio). 19(5):408-18.
- Hattori K, Dias S, Heissig B, Hackett NR, Lyden D, et al. (2001)Vascular endothelial growth factor and angiopoietin-1 stimulate postnatal hematopoiesis by recruitment of vasculogenic and hematopoietic stem cells. J Experiment Med. 193(9):1005-14.
- Hiroshi M, Michael DC, John B, Judah AD. (1988) Nerve growth factor promotes human hemopoietic colony growth and differentiation. Proceedings of the National Academy of Sciences-PNAS. 85(17):6508-12.
- Yang X, Tare RS, Partridge KA, Roach HI, Clarke NM, et al. (2003) Induction of human osteoprogenitorchemotaxis, proliferation, differentiation, and bone formation by osteoblast stimulating factor-1/pleiotrophin: Osteoconductive biomimetic scaffolds for tissue engineering. Journal of Bone and Min Res. 18(1):47-57.
- Karp JM, LengTeo GS. (2009)Mesenchymal stem cell homing: The devil is in the details. Cell Stem Cell. 4(3):206-16.
- Kim J, Kang J, Park J, Choi Y, Choi K, et al. (2009) Biological characterization of long-term cultured human mesenchymal stem cells. Arch Pharm Res. 32(1):117-26.
- Djouad F, Plence P, Bony C, Tropel P, Apparailly F, et al. (2003) Immunosuppressive effect of mesenchymal stem cells favors tumor growth in allogeneic animals. Blood. 102(10):3837-44.
- Tolar J, Nauta AJ, Osborn MJ, Panoskaltsis MA, McElmurry RT, et al. (2007) Sarcoma derived from cultured mesenchymal stem cells. Stem Cells (Dayton, Ohio). 25(2):371-9.
- Aguilar S, Nye E, Chan J, Loebinger M, Spencer-Dene B, et al. (2007) Murine but not human mesenchymal stem cells generate osteosarcoma-like lesions in the lung. Stem Cells (Dayton, Ohio. 25(6):1586-94.
- Das M, Mayilsamy K, Mohapatra SS, Mohapatra S. (2019)Mesenchymal stem cell therapy for the treatment of traumatic brain injury: Progress and prospects. Rev Neurosci. 30(8):839-55.
- Tian C, Wang X, Wang X, Wang L, Wang X, et al. (2013)Autologous bone marrow mesenchymal stem cell therapy in the subacute stage of traumatic brain injury by lumbar puncture. Experiment Clinic Transplant. 11(2):176-81.
- Hrbacek F, Knazkova M, Nyvlt D, Laska K, Mueller CW, et al. Active layer monitoring at CALM-S site near J.G. mendel station, james ross island, eastern antarctic peninsula. Science of the Total Environment. 601-602:987-97.
- Vaquero J, Zurita M, Rico MA, Bonilla C, Aguayo C, et al. (2017) Repeated subarachnoid administrations of autologous mesenchymal stromal cells supported in autologous plasma improve quality of life in patients suffering incomplete spinal cord injury. Cytotherapy (Oxford, England). 19(3):349-59.
- Zhang Z, Guan L, Zhang K, Zhang Q, Dai L. (2008) A combined procedure to deliver autologous mesenchymal stromal cells to patients with traumatic brain injury. Cytotherapy (Oxford, England). 10(2):134-9.
- Park JH, Kim DY, Sung IY, Choi GH, Jeon MH, et al. (2012) Long-term results of spinal cord injury therapy using mesenchymal stem cells derived from bone marrow in humans. Neurosurg. 70(5):1238-47.
- Milczarek O, Jarocha D, Starowicz-Filip A, Kwiatkowski S, Badyra B, et al. (2018) Multiple autologous bone marrow-derived CD271+ mesenchymal stem cell transplantation overcomes drug-resistant epilepsy in children. Stem Cells Translat Med. 7(1):20-33.
- Hlebokazov F, Dakukina T, Ihnatsenko S, Kosmacheva S, Potapnev M, et al. (2017) Treatment of refractory epilepsy patients with autologous mesenchymal stem cells reduces seizure frequency: An open label study. Adv Med Sci. 62(2):273-9.